© Springer International Publishing Switzerland 2017
Norbert Weidner, Rüdiger Rupp and Keith E. Tansey (eds.)Neurological Aspects of Spinal Cord Injury10.1007/978-3-319-46293-6_2525. Translation: Relevance of Spinal Cord Injury Animal Models
(1)
International Collaboration on Repair Discoveries, Blusson Spinal Cord Centre, 818 West 10th Avenue, Vancouver, BC, Canada, V5Z 1M9
(2)
Department of Orthopaedics, University of British Columbia, International Collaboration on Repair Discoveries (ICORD), 6th Floor, Blusson Spinal Cord Center, VGH 818 West 10th Avenue, Vancouver, BC, Canada, V5Z 1M9
Abstract
There are currently no therapeutic interventions available for the treatment of spinal cord injury (SCI). The discovery and validation of the growing number of promising therapies will require continued reliance on preclinical animal models of SCI prior to human translation. Animal models of SCI are instrumental in better understanding the mechanisms involved in traumatic SCI and evaluating the efficacy of therapeutic interventions. Over the past 40 years, substantial gains have been made in developing consistent, reproducible and reliable animal SCI models.
These models vary in terms of the species utilized, injury location, and injury mechanism, each with its own advantages and disadvantages. While the controlled experimental environment of preclinical studies is considered advantageous, it is this that makes animal models distinct from clinical reality, where there is considerable heterogeneity in baseline health and injury mechanics. The challenge then, is to evaluate what level of preclinical evidence is sufficient to proceed with clinical trials.The range of experimental paradigms available to the scientific community give new opportunities to address translational questions prior to human testing. Continued open communication involving scientists, clinicians, regulatory agencies, funding agencies, and the individuals living with SCI is required to move forward efficiently towards the establishment of novel therapeutics for the treatment of SCI.
25.1 Introduction
Each year, more than 10,000 individuals in North America and many thousands more around the globe are paralyzed after sustaining an acute spinal cord injury (SCI) (see chapter 1), leaving them to endure one of the most physically and psychologically devastating injuries known to mankind. More than four decades of passionate research in this field have generated substantial progress in understanding the mechanisms that underlie SCI pathology. Alongside this, a growing number of therapeutic interventions have shown promise in animal models of SCI and are vying for human translation [81].
While the optimism surrounding clinical translation reflects significant scientific progress, there are also important challenges that extend beyond the decision to move a promising experimental therapy ‘from bench to bedside’. While a handful of drug therapies have emerged from the laboratory to be tested in humans with acute SCI, none have succeeded in demonstrating convincing neurological benefit in large-scale clinical trials [26, 57, 82, 97]. These past clinical trials in acute SCI have highlighted the significant time and tremendous resources required for completion. For example, the clinical evaluation of Sygen for acute SCI occurred over a 14-year period from the initiation of a phase I study to the completion of its multicentre phase III randomized trial [31, 32] and required the collective efforts of over 20 neurotrauma centres in North America. The heterogeneity of human SCI necessitates the recruitment of large numbers of patients for adequately powered clinical trials, which is challenging due to the relatively low incidence of traumatic SCI (see chapter 20).
Given the considerable time, financial, and personnel resources required to complete a clinical trial, it is hard to argue against the need for demonstrating robust efficacy of a therapy in preclinical studies prior to embarking upon a clinical trial. But how much is enough? Does the animal species utilized for such experiments matter? Does the injury paradigm matter? What preclinical pieces of evidence that a therapy actually ‘works’ need to be assembled to establish a persuasive rationale to move forward with human translation? While efforts have been made to create an objective method for evaluating the ‘translational readiness’ of potentially promising SCI therapies [53], there are no widely agreed upon criteria to determine when a treatment has received enough preclinical evidence to justify large-scale clinical trials. In part, this is related to the fact that we do not have examples of treatments proven to be efficacious in human trials that then enable us to look back at how they were evaluated preclinically. In the absence of such guidance, the field depends on animal models of SCI to evaluate the robustness of therapies prior to human translation, with the hope of improving the likelihood of success in clinical trials.
25.2 History of Translation in SCI
Clinical trials of interventions that aim to improve neurological function after SCI can take many years to complete [25, 56, 87, 97, 98] and cost an enormous amount of money, as recently illustrated by Geron Corporation’s decision to prematurely terminate their embryonic stem cell trial [29] (see chapter 21). While the SCI community absorbs these direct costs of the clinical trial, it also bears the potential opportunity costs of being unable to evaluate other (possibly even more promising) treatments because of limited money, patients, and clinical research centres with the capacity to conduct acute SCI trials. Additionally, while individuals with SCI who participate in an early (phase I or IIa) experimental trial might acknowledge that the trial’s focus is to confirm the safety of the therapy being tested, it is understandable that they invariably hope that it will be of some benefit to them [49]. It is therefore the responsibility of the research community to ensure that the decision to move a treatment forward into clinical trials is based on careful consideration of its supporting preclinical evidence in experimental animal models [24].
25.3 Animal Models of SCI
Animal models allow for the controlled and rigorous study of injury responses and recovery. The animal cohorts can be relatively homogenous, the injury characteristics and conditions can be precisely controlled, and – compared to human clinical trials – the experiments are relatively inexpensive. In addition to tightly controlled experimental conditions, animal models offer the obvious advantage of being able to harvest the injured spinal cord itself from the euthanized animal for a myriad of different analyses (e.g. histology, biochemistry, molecular biology). It is important to recognize that the same factors that are often touted as advantages of animal model studies – tightly controlled conditions, homogeneous cohorts, precisely delivered mechanical injuries – also make such experiments quite distinct from clinical reality. In the latter, there is considerable heterogeneity and variability in baseline health, injury biomechanics, and subsequent treatment. This is a typically under-appreciated limitation of experimental paradigms that employ animal models for SCI research.
25.3.1 Injury Models
Contusion Models
The majority of traumatic SCIs are caused by blunt trauma related to motor vehicle accidents (43 %), falls (18.8 %), and sporting injuries (11.1 %). These involve a sudden contusive force being applied to the spinal cord as the surrounding bony/ligamentous spinal column fails [75]. Penetrating trauma to the spinal cord from gunshot wounds or knife injuries is relatively uncommon in Western societies (see chapter 1).
The earliest forms of SCI modelling involved a replication of this contusive form of injury. The first documented animal model of SCI was described in 1911 by Alfred Reginald Allen [3]. In this canine SCI model, a 30 g mass was dropped from an 11 cm height along a rod, onto the dorsal surface of the exposed spinal cord. Generally speaking, the contusion injury models currently utilized are modifications of the original Allen injury model [100]. The first widely used weight-drop SCI contusion device in rodents was the New York University impactor [35]. Injury severity with the NYU impactor is adjusted by altering the height from which a 10 g rod is dropped onto the exposed thoracic spinal cord: 6.25 mm (mild), 12.5 mm (moderate), 25 mm (severe), and 50 mm (very severe). The rod velocity is monitored to allow for validation and standardization of the injury mechanics. The NYU impactor was used in the ‘multicenter animal spinal cord injury study’ or ‘MASCIS’ and is often referred to now as the NYU-MASCIS or MASCIS impactor.
The NYU impactor was followed by the development of an electromagnetic impactor device by the Ohio State University (OSU) Spinal Cord Research Center. This device was computer controlled to precisely deliver a defined displacement to the dorsal thoracic spinal cord surface [11, 16, 43, 91]. The OSU electromagnetic SCI device (ESCID) was originally designed for use with rats but was also modified to be applicable in mice as well [43]. It has also been used to model cervical spinal cord injuries [61]. The computer control allows for the adjustment of displacement and velocity and provides feedback on the maximal impact force delivered.
In 2003, Scheff et al. reported on the ability of a new device, the infinite horizon (IH) impactor, to produce graded morphological and behavioural changes in a rodent model following contusion injury to the spinal cord at T10 [84]. To control the injury severity with the IH impactor, the impact force (kdyne) is adjusted; this is in contrast to the NYU-MASCIS impactor and OSU impactor in which the injury severity is adjusted by height of weight drop (and conversely velocity) or depth of tissue displacement (mm), respectively. The impactor has been modified to induce unilateral cervical spinal cord injuries [62] and can also be modified for use in smaller rodents such as mice by replacing the impacting tip with a smaller version [14]. A technical challenge with the IH impactor is in grasping the spinal column securely with its clamps. Streijger et al. recently reported on a design for a custom clamping system to improve upon this [93]. Like the NYU-MASCIS impactor, these contusive models are able to create graded injuries within the spinal cord and are characterized by central haemorrhagic necrosis, ischaemia and inflammation [12].
Clip-Compression Models
In 1978, Rivlin and Tator developed the ‘clip-compression’ model of SCI in rats, in which the spinal cord was compressed dorsoventrally between the arms of a modified aneurysm clip [83]. The clips can be calibrated to exert a specific force to the spinal cord and induce graded injury severities [80]. This model demonstrated the relationship between the severity of neurologic injury and the severity and duration of compression. The Rivlin-Tator aneurysm clip was originally designed for use in the thoracic cord [83]. Recently, Fehlings and colleagues have developed modified protocols for its use at the C6–C7 and C7–T1 spinal levels [28].
The clip-compression model aims to replicate the effect of persistent spinal cord compression that is commonly found in clinical SCI. Compression, however, is typically applied for 1 min, which is vastly different from the duration of persistent compression that is witnessed in human SCI. The clip-compression and contusion injuries both impart a blunt force to the spinal cord. They differ in that clip-compression injury lacks the initial velocity of the contusion injury, while contusion injuries do not impart the sustained spinal cord compression observed in human SCI. Even for commercially available contusion impactors, the velocity with which the spinal cord is impacted is considerably lower than what is estimated to occur in human SCI [77, 104].
Balloon-Induced Compression Models
Tarlov first proposed the balloon-induced compression injury model in 1953 [96]. The model was developed due to its simplicity and non-invasive nature. Initially, a small hemilaminectomy defect was created to access the epidural space, into which a Fogarty catheter was inserted and advanced cranially to one or two higher spinal levels [67]. Alternatively, the catheter may be installed in the lumbosacral spine and advanced through the epidural space to the thoracic spinal cord. Inflating the balloon at the catheter tip with saline imparts the compressive force against the spinal cord [59]. Again, the injury can be graded using varied volumes within the balloon. This method of injury has been used in a variety of large animal species, including canines and monkeys [36, 59].
Transection Models
While full transections are rare in the clinical setting [78, 94], the transection models offer the advantages of investigating axonal regeneration/plasticity, degeneration, tissue engineering strategies and cell transplantation [12]. Transections can be either complete or incomplete, with the latter intended to precisely interrupt specific tracts [18, 90]. For example, a dorsal hemisection can be used for selective transection of the corticospinal tracts, while a dorsolateral quadrant lesion can be used to transect the rubrospinal tract [12, 94]. The key advantage to transection models (either full or partial) is the more clear interpretation of axonal regeneration studies.
Photochemical Models
A photochemical-based model of SCI was first developed in 1986 [101] and has been used as a method of creating a discrete SCI without significant primary trauma [30]. A dye is infused systemically and then activated within a discrete area of the spinal cord using an argon laser. The resultant photochemical reaction produces single oxygen molecules on the endothelial surface of spinal cord vessels, which triggers an aggressive platelet response, vessel occlusion, and damage to the parenchymal tissue [37]. The model is considered reliable and reproducible and does not require a full laminectomy. While this model is quite distinct from the high-energy injury models that resemble human injury, it can be useful for studying features of the secondary injury phase in the absence of significant mechanical trauma.
Excitotoxic Models
Injury in the excitotoxic model is induced by an intraspinal or intrathecal injection of excitotoxins such as quisqualic acid, an α-amino-3-hydroxy-5-methyl-4-isoxazolepropionic acid (AMPA)-metabotropic receptor agonist [106]. These excitotoxins are particularly useful for generating rodent models of neuropathic pain with thermal hyperalgesia and mechanical allodynia [64, 71].
25.3.2 Animal Species Used in Modelling SCI
In addition to the variety of injury mechanisms used to induce SCI, there are numerous animal species that are used for SCI research. Rats and mice are by far the most commonly used animals for in vivo SCI modelling and experimentation [7, 33]. Other experimental species used for SCI research include gerbils, guinea pigs, hamsters, cats, dogs, pigs, and non-human primates [17, 42, 60, 67, 72, 76, 86, 108]. Other models include invertebrates such as eels [94], whose unique regenerative capacities have been investigated in an effort to understand the regenerative processes and how they might be harnessed in humans. While there is a breadth of animal species available for SCI modelling, one should recognize that each has its own advantages and disadvantages, and none necessarily represent the human condition with perfect biofidelity.
Rat
Rat models are the most commonly used for SCI experimentation, and most SCI models were initially described and characterized in the rat [15, 27, 84, 91, 95, 105, 107]. They are relatively inexpensive, easy to handle and care for, and have long-established injury mechanisms available (e.g. contusion, clip compression, transection). They have well-described neuroanatomy, and there exists a wide array of behavioural tools as outcome measures. Locomotor recovery is typically evaluated with the widely used Basso, Beattie, and Bresnahan (BBB) scale, which has the advantage of being relatively inexpensive and comparable across laboratories [9]. More sophisticated systems for testing locomotor recovery have been established and are commercially available (e.g. catwalk) [99, 107]. While traditionally the rodent SCI models involved a mid- or low-thoracic injury and an assessment of lower limb recovery, a number of groups have developed methods for injuring the cervical spinal cord to specifically evaluate forelimb responses [40, 63, 69, 70, 85, 103].
Mouse
Mouse models of SCI have the significant advantage of enabling the application of sophisticated genetic/molecular techniques to investigate the role of specific genetic factors that may influence biological responses to injury (e.g. cell death or axonal regeneration). The ability to enhance or delete specific genes allows for the study of their roles in gain of function and loss of function experiments. Using mice with a specific gene deletion has become a standard approach in SCI research, and Cre-lox technology along with increasing numbers of transgenic mice has provided greater spatiotemporal control of the knockout strategy and a better understanding on specific factors that affect axonal outgrowth. The small size of mice, however, makes surgical procedures more difficult and prohibits many device implantations [71, 94]. Similar to rats, investigators have modified the traditional thoracic SCI approaches to study cervical SCI in mice [1, 47, 92].
Cat
The cat has been used extensively in locomotor studies that have contributed to a greater understanding of spinal networks controlling and regulating locomotor activity. The larger size of cats allows for a wider range of surgical implementation and the opportunity to execute detailed kinematic analyses in locomotor and postural studies. Anatomically, the spinal cord of cats is organized similarly to humans – specifically, the main part of the corticospinal tract is located in the dorsolateral spinal cord, while in rodents it is located below the dorsal columns. In the early twentieth century, the demonstration that the spinal cord could generate the basic rhythm of locomotion by itself was first shown in an adult cat model of SCI [19]. The cat SCI model has served as the foundation for seminal insights into locomotor training by researchers such as Serge Rossignol [6], Reggie Edgerton [68], and Susan Harkema [38]. The evidence of an intrinsic spinal locomotor network that can be activated using different types of stimulation was important for the development of rehabilitation strategies for spinal cord injured humans.
Dog
Within the last two decades, rodent models of SCI have largely replaced the use of dogs in experimental SCI research. However, there has been increasing interest in ‘naturally occurring’ SCI that occurs in domestic dogs [44]. Dogs naturally suffer spinal cord injuries as the result of traffic accidents, or – in some species – acute thoracolumbar disc herniations [66]. Because of this, the mechanisms of injury are often similar to human SCI: vertebral fracture-luxation and disc extrusions, which both produce a combination contusion-compression lesion to the ventral aspect of the cord – something that is difficult to model experimentally [44]. The paradigm of a ‘naturally occurring’ SCI (in contrast to an experimentally induced SCI) lends itself to the conduct of a true ‘clinical trial’ that resembles how human trials are conducted [34]. Essentially, the clinical population of domestic dogs with SCI forms a ‘surrogate human’ population of clinical patients in which to test the efficacy of potential treatments for SCI. Recently, a population of spinal cord injured dogs was used in a prospective randomized clinical trial of an intraspinal transplantation of glial cells derived from the olfactory mucosa [34]. This study demonstrates the potential utility of this naturally occurring SCI in dogs to conduct a clinical trial of a novel therapeutic much in the same way as it would be conducted in humans.
Pig
The pig has the spinal cord and CSF dimensions that are more similar than rodents to those of an adult human [60]. The obvious differences in size and anatomy and potentially greater similarities in biological responses to injury between humans and higher-order animals make a porcine model of SCI a useful model for the investigation of biological and cellular transplantation studies [60]. Additionally, while the costs associated with conducting studies in large animals are certainly higher than those with rodents, they are a fraction of those associated with primate studies. A number of investigators have described the development of pig models of SCI using a weight-drop impactor, computer-controlled contusion/compression devices, or calibrated vascular clips [60, 72, 108]. Using a weight-drop contusion device, Lee et al. developed a porcine model of traumatic thoracic SCI in Yucatan miniature pigs, where varying degrees of injury severity can be induced by altering the height (5, 10, 20, 30, 40, and 50 cm) from which a 50 g weight is dropped onto the dorsal aspect of the T10/T11 segment [60]. Locomotor recovery was evaluated using their Porcine Thoracic Injury Behaviour Scale (PTIBS), which was sensitive at distinguishing recovery amongst animals of different injury severities [60]. Using a computer-controlled system, Navarro et al. developed a chronic SCI model in adult Gottingen-Minnesota pigs, where they showed consistent development of paraplegia following a 2.5 kg compression force, delivered at a velocity of 3 cm/s on the dorsal aspect of the T12 segment [72]. Zurita et al. developed a vascular clip model of SCI in adult minipigs, where paralysis was caused by the epidural application of two vascular clips for 30 min [108].
Non-human primate
Non-human primate studies are primarily limited by their specialized facility requirements and high cost related to the intensity of animal care. Some also have ethical objections to their use. Nevertheless, their ‘relatedness’ to humans has made them an appealing species to preclinically evaluate novel therapies and has motivated researchers to develop injury models in them. In 2012, Nout et al. reported on a lateral hemisection model of cervical SCI in adult rhesus monkeys [76]. A lateral hemisection allows for precise control over the lesion location and extent, and it allows for some preservation of function in experimental animals so that the injury morbidity is reduced. A cervical contusion model of SCI in marmosets was developed by Iwanami et al., where a 17 g weight is dropped from a height of 50 mm onto the exposed dura matter of the C5 segment [42].
One of the main advantages of a cervical SCI model is the similarity in upper extremity function between humans and non-human primates. As regaining hand function is the highest priority for tetraplegic individuals [4], evaluating therapies in a primate cervical SCI model that influences the recovery of hand function is of translational importance [76]. Demonstrating the restoration of hand function in a primate model of cervical SCI is an important consideration for the translation of invasive and inherently risky cell transplantation therapies.
25.3.3 Limitations of Preclinical Experimentation Using Animal Models
While much effort has been made to refine various animal models in different injury species, it is worth at least acknowledging some of the broad limitations of the experimentation paradigms utilized in preclinical studies. As mentioned earlier, human SCI is incredibly heterogeneous in its causes, consequences, and pathology. Conversely, in animal studies, researchers typically endeavour to minimize experimental variability in as many ways as possible. Animals of the same weight, age, gender, and, sometimes, genetic background are accrued for an experiment, then subjected to a precise biomechanical injury (which imperfectly simulates the high velocity with which human injury occurs), and subsequently housed in identical post-injury conditions.
Additionally, conditions inherent to the experiment may influence the outcome. Anaesthesia, for example, is essential for the experiment but may by itself has neuroprotective effects. Pentobarbital and isoflurane, commonly used anaesthetics for SCI experiments, have been shown to reduce infarct volume following ischaemia [39, 41, 46]. The general anaesthetic, ketamine, has been shown to reduce functional and histopathological indices of injury in gerbils subjected to cerebral ischaemia [39]. While there is no alternative to appropriately anaesthetizing the animals in experimental injury models, the potential effects of these drugs (albeit distributed equally across an experiment) need to be acknowledged.
It should also be acknowledged that the functional outcomes and behavioural assessments differ from experimental models and the clinical setting. Much effort has gone into the design of assessment scales and procedures that accurately measure functional recovery in animals. Some of the most commonly used tests in SCI research include the BBB scale [8], the Tarlov open-field test [96], and the inclined plane test [83]. Both the Tarlov and inclined plane tests assess general locomotor ability and do not reflect specific changes in motor or sensory function [48]. Alternatively, the BBB scale emphasizes hind limb function and does not assess other movements, which require coordinated spinal cord activity [2]. In the end, despite the refinements and widespread use of such outcome measures, the reality is that these measures have no physiologic correlate in humans. For example, it is impossible to predict how the achievement of ‘plantar weight-supported stepping’ on the BBB scale would translate into human lower extremity function. Researchers are warned to resist the temptation to extrapolate such changes in animal behaviour to human locomotor recovery.
Finally, the SCI field has become increasingly aware of the challenge of demonstrating the robustness of its novel therapies, as manifested by the difficulty in independently reproducing promising experimental findings (see chapter 20 and 21). This has, to some extent, been recognized for decades in many areas of biomedical research [22, 23, 88]. Recently, the biotechnology industry has reported on the difficulty of replicating many promising findings published in peer-reviewed journals [10, 79]. Poor study design and reporting practices in SCI research have likely resulted in biased results, considerable waste, and arguably, a slowing of progress towards developing effective therapeutics [65]. In the SCI field, a formal replication initiative funded by the National Institute of Neurological Disorders and Stroke (NINDS) resulted in the failure of the majority of promising SCI therapies [89]. Factors like litter-to-litter variability can impact behavioural studies significantly [58]. Concerns have also been raised regarding a number of issues in neuroscience publications, including inappropriate statistics [20, 73], low power [21], and a need to decrease p values from 0.05 to 0.005–0.001 [45]. Recently there has been support for implementing reporting standards for preclinical research that are analogous to the reporting of clinical trials, as the absence of such standards may influence the interpretation of study results [65]. For example, RhoA/Rock inhibitor and stem cell studies for SCI treatment have been shown to describe more favourable outcomes when the articles do not report whether investigators were blinded during behavioural testing [5, 102]. Efforts such as these will improve the quality of SCI research and help the field interpret the overwhelming body of literature that is emerging on novel therapeutics.
25.4 What Constitutes ‘Enough’ Preclinical Evidence to Proceed with Human Trials?
The experience of testing promising experimental therapies in clinical trials of SCI has taught the field much about the challenges associated with successfully translating such novel technologies into clinically efficacious treatments for human patients. These experiences have led to important initiatives to identify the obstacles and challenges to successful translation and potentially improve upon the chances of succeeding in future trials [25, 56, 87, 98]. Such initiatives span the continuum from recommendations around preclinical laboratory studies to the planning and conduct of clinical trials.
One area of specific interest within this continuum is the issue of determining what preclinical evidence is needed to justify moving a promising experimental therapy towards a lengthy and expensive human clinical trial [13, 24]: When demonstrating a therapy’s efficacy, what animal model is sufficient? What is the most relevant injury model of human SCI? What constitutes ‘clinically meaningful efficacy?’ How much scientific evidence that a cellular therapy ‘works’ in preclinical models of SCI is enough before proceeding with a human clinical trial? These are complex questions which do not currently have straightforward answers, as we do not have the luxury of looking back retrospectively at how clinically efficacious therapies for SCI were evaluated preclinically prior to human testing. On one hand, the devastating effects of SCI and the untreatable nature of the neurologic impairment provide good reasons to aggressively move therapies forward that appear promising, particularly if they have an acceptable safety profile. On the other hand, the time and money required to complete a clinical trial and the potential risks associated with some therapies such as cellular transplantation provide good reason to ‘thoroughly investigate and establish the robustness’ of a promising therapy in preclinical studies.
We have undertaken a series of initiatives to characterize the perspectives of various stakeholders on this issue and provide the field with some guidance around these translational considerations [49–51, 53–55]. We first conducted a survey of the research community about what they felt was needed to translate a novel therapy to human clinical trials [51]. This survey was completed by over 300 respondents and provided a wide breadth of opinions from scientists, clinicians, and trainees. The results of this survey revealed a number of interesting perspectives around translation. There was strong support for testing therapies and demonstrating efficacy in multiple animal species (in addition to rodents) before human clinical trials. Particularly for invasive cell transplantation strategies, the majority advocated for large animal and primate model testing prior to human trials. The majority of respondents favoured contusion injuries over compression injuries as being the most clinically relevant mechanism of injury. Respondents were also keen to see therapies preclinically tested in relevant post-injury time windows, recognizing that there is an inevitable delay in administering treatments in the human setting. There was nearly universal support for the need for independent replication.
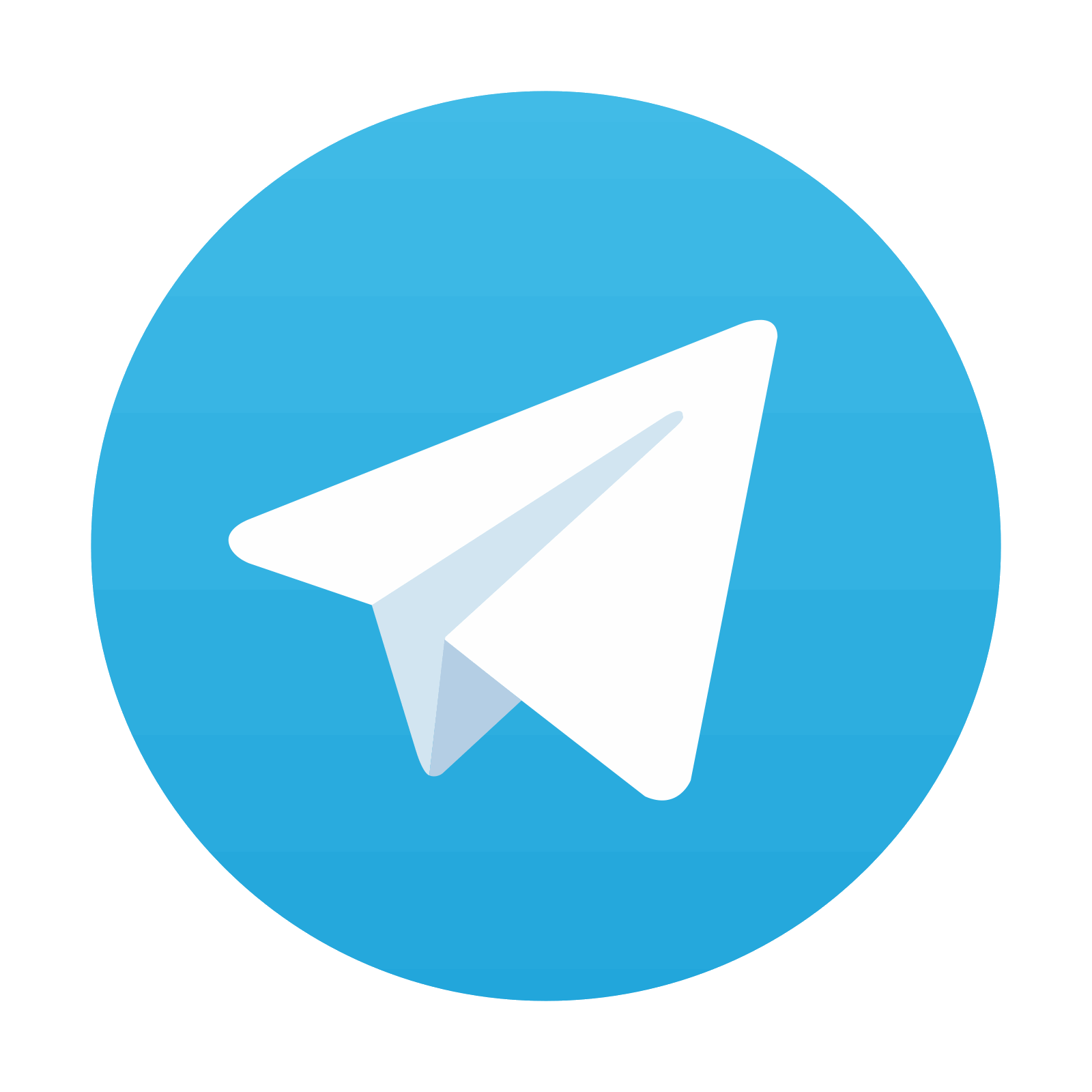
Stay updated, free articles. Join our Telegram channel
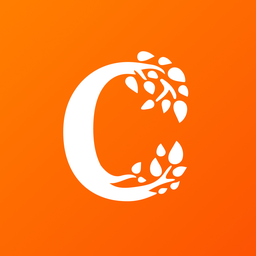
Full access? Get Clinical Tree
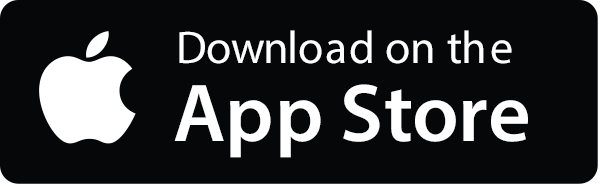
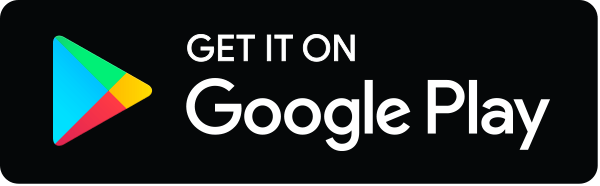