Fig. 5.1
Electromyographic (EMG) activities in lower limb muscles (TA tibialis anterior, MG medial gastrocnemius, RF rectus femoris, BF biceps femoris) in a human subject while walking comfortably. Calibration bars represent 100 μV and 500 N (vertical), and 1 second (horizontal), respectively
The emergence of these motor patterns takes place automatically and does not necessarily accompany one’s intention. Indeed, we can walk or run while talking with a friend. When dashing to catch a baseball, our attention is directed toward the ball or the forthcoming action after catching the ball rather than on the details of the ongoing running movement itself. How, then, are these highly coordinated movements possible with little or no conscious awareness? We next describe the neural mechanisms responsible for generating the motor output based on basic studies both in animals and humans.
5.3 Central Pattern Generators: CPGs
5.3.1 Overview and Direct Evidences from Animal Studies
It is well known that human neonates exhibit stepping-like movements in their lower extremities when the center of body mass is moved forward by external support (Yang et al. 1998). Cats that have undergone surgical decerebration show stepping-like hindlimb movements in accordance with the treadmill movement underneath them (Barbeau and Rossignol 1987). These behavioral responses clearly indicate that the initiation of locomotory behavior does not require a contribution of the higher structures in the brain. Rather, as much research has shown, there exist functional networks in the spinal cord that are specialized for executing locomotory movements and play a significant role in generating rhythmic motor patterns. These functional networks are referred to as “central pattern generators” (CPGs) and consist of sets of interneurons in the spinal cord.
The original concept of spinal CPGs was probably first addressed in a study by Sherrington (1910), and was closely followed by work by Brown (1911) who, using a combination of low spinal transection and de-afferentation in a cat preparation, demonstrated the existence of a movement control circuit in the lumbar region of the spinal cord. In the last several decades, such neural mechanisms have been extensively and systematically studied in both invertebrates (for example, locust flight, Wilson 1961) and vertebrates such as swimming in the lamprey (Grillner et al. 1981; Wallén and Williams 1984) and walking in the cat (Grillner and Zangger 1979). In one example, Wallén and Williams (1984) obtained bilateral electromyographic recordings from two different segments in the spinal cord of an intact lamprey. The results, as expected, showed rhythmic activity, with a slight time lag between the segments and alternating patterns with a lack of co-activation contralaterally. More surprising and intriguing was the result that similar neural activity was evident even under fictive locomotion with an isolated spinal cord. That is, the rhythmic motor patterns were maintained without any external input. In earlier work, patterned activities had even been identified in an isolated in vitro spinal cord utilizing pharmacological intervention (Cohen and Wallén 1980). From these results and others, CPGs were understood to be neural networks being capable of the formation of rhythmic patterns, even in absence of external inputs such as those from the brain or the periphery (Dietz 2002).
5.3.2 Possible Existence of CPGs in Humans
Despite the considerable body of evidence for the existence of CPGs in animals, the possibility of its existence in humans has been a long-disputed question. The presence of CPGs in humans has become evident only after studies by Calancie et al. (1994) and subsequently by Dimitrijevic et al. (1998) in spinal-cord injured patients. With a chronic, complete severing of the spinal-cord (C5 at the highest and T8 at the lowest in the injury site) with patients lying in a supine posture, continuous epidural stimulation was delivered to the lumbosacral region with a constant frequency (Dimitrijevic et al. 1998). The result clearly showed that, despite the trained stimulation, phasic locomotor-like EMG activities as low as 4 Hz (in the stepping frequency) were evident when the stimulation was delivered at a certain frequency (mostly between 25 and 50 Hz). The results were later supported by Gerasimenko et al. (2010) using less invasive experimental procedures. Electromagnetic stimulation applied to the spinal cord in the lower thoracic region (T11-T12) from the surface of the skin resulted in the emergence of involuntary stepping-like movements in the lower limb among non-injured subjects while lying in the decubitus position and relatively free from gravitational forces. The movements were further enhanced by the addition of vibration to the leg muscles (mimicking proprioceptive input). These results combined suggest the existence of CPGs in humans, just as was found in the animal models utilized in the earlier studies. From these studies it is also clear that, minimally, CPGs in humans are located in the lower region of the spinal cord.
Other studies have addressed the location of CPGs in the spinal cord of humans. Dietz et al. (1999) compared locomotory function in chronic spinal-cord injured patients with different injury sites to that of normal subjects. Deficits in locomotory function took place in a phased manner depending on the injury level rather than a major, stepwise change at a particular level (Dietz et al. 1999). CPGs were therefore shown to be located over a wide range in the spinal cord (up to the cervical level) and not necessarily localized in the lower regions. The fact that CPGs are located as high as the cervical level may be linked to recent results showing that upper limb movements during locomotion are also under the control of CPGs (for review, see Zehr and Duysens 2004). This higher location was demonstrated on the basis of phase dependent modulation and reflex reversal in lower limb cutaneous reflex responses during arm cycling (Zehr and Kido 2001).
5.4 Influence of Supraspinal and Afferent Input
Execution of locomotion movements is known to be affected by both supraspinal inputs and inputs from the periphery. Schubert et al. (1997) investigated a possible contribution of the corticospinal tract using transcranial magnetic stimulation (TMS). By applying the magnetic stimulation to the motor cortex under different phases of normal walking, they revealed that responses in the ankle flexor TA and extensor MG muscles were modulated in a phase-dependent manner, independently of the background EMG level. Moreover, the degree of facilitation relative to the amplitude of the evoked potentials during tonic contraction of the muscles was larger in the TA than in the MG muscle, thus showing a difference in the contribution of corticospinal pathways to flexors and extensors. A recent study further revealed that phase-dependent modulation of corticospinal excitability took place even under passive stepping induced by use of a robotic driven-gait orthosis (Kamibayashi et al. 2009). Since the latter result was not observed during the same passive stepping task if it was executed in air, the authors concluded that load-afferent input played a significant role in the phase-related modulation of corticospinal excitability.
A similar phase-related modulation was observed in the excitability of spinally-mediated reflex pathways. Nakajima et al. (2008) demonstrated that the cutaneous reflex in the TA muscle was modulated in the same way in a normal gait as when walking passively. Despite a constant background EMG level, the response was prominently enhanced only under particular phases where the activity of the particular muscle was essential for normal walking such as at the end of the stance phase and at the beginning of the swing phases. The modulation however, was not evident when the stepping movement was executed in the air (thus with no loading). Using the monosynaptic H-reflex, Kamibayashi et al. (2010) showed similar phase-dependent modulation. These last two studies demonstrate that afferent input is also important in shaping locomotion related motor output.
5.5 Plasticity in the CNS and Its Specificity
In addition to the relatively automatic mechanisms involved in the control of locomotory movements, the mammalian CNS also has the potential to undergo plastic reorganization in response to particular stimuli. This reorganization involved both anatomical and functional alterations (Raineteau and Schwab 2001). Examples of the former are lesions of neural pathways and their reconnection through sprouting while the latter is demonstrated by functional changes in pre-exiting pathways which occur and include changes in the efficacy of synaptic transmission and level of excitability of the various pathways (for review, see Zehr 2006; Wolpaw 2007). This plastic property of the CNS is an important part of what is addressed by training procedures that are designed to improve motor performance.
It has been demonstrated that functional alterations can take place even in the simplest behavior of the CNS, spinally mediated monosynaptic reflexes. Operant conditioning in monkeys (Wolpaw et al. 1986), rats (Chen and Wolpaw 1995), mice (Carp et al. 2006), and recently in humans (Thompson et al. 2009) provides clear evidence of this alteration. Subjects underwent long term training (several weeks to months) requiring them to either increase or decrease their reflex amplitude. Rewards of juice or money were provided on the basis of their success. Weeks or months after the initiation of the training, the reflex responses were successfully enhanced or depressed in accordance with the requirement for gaining a reward. Since the responses were those obtained under a constant background EMG level, it is considered that some functional reorganization took place within the neural system.
Along with the above mentioned examples, in humans particularly, evidence for functional alternations in the CNS have been shown by cross-sectional comparisons among populations with different physical backgrounds. For example, a comparison of the amplitudes of the electrically induced Hoffmann (H-) reflex between elite ballet dancers and ordinary well-trained athletes clearly showed a suppressed response in the ballet dancers (Nielsen et al. 1993). Similarly, another study demonstrated that the amplitude of the H-reflex in comparison to that of sedentary subjects was smaller in sprinters and greater in endurance runners (Maffiuletti et al. 2001). These results clearly show that not only the amount but also the type of physical activity has an influence on the functional alteration that is made. Thus, functional reorganization is very context specific.
5.6 Specificity in Locomotion-Related Neural Mechanisms
5.6.1 Direct Evidence from Animal Studies
Given the specificity of functional alterations in particular neural pathways, a question arising next upon construction of locomotion training is the possible specificity in the neural mechanisms underlying locomotion movements. Recent studies focusing on swimming behavior in larval zebrafish demonstrated specificity in the spinal interneurons that were recruited depending on swimming modes with different frequencies (McLean et al. 2007, 2008). For reference, it was previously demonstrated that zebrafish have mainly two different modes (one with low frequency to routinely swim and another with higher frequencies for quick movements) (Budick and O’Malley 2000). McLean et al. (2008) showed that recruitment of the spinal interneurons occurred depending on specific swimming frequencies while that in motoneurons took place only incrementally following classic size principle. That is, there was certain independence in the neural mechanisms responsible for different swimming modes. From a phylogenetic perspective, it is highly possible that the mechanisms underlying the basic function such as locomotion are shared in humans as well.
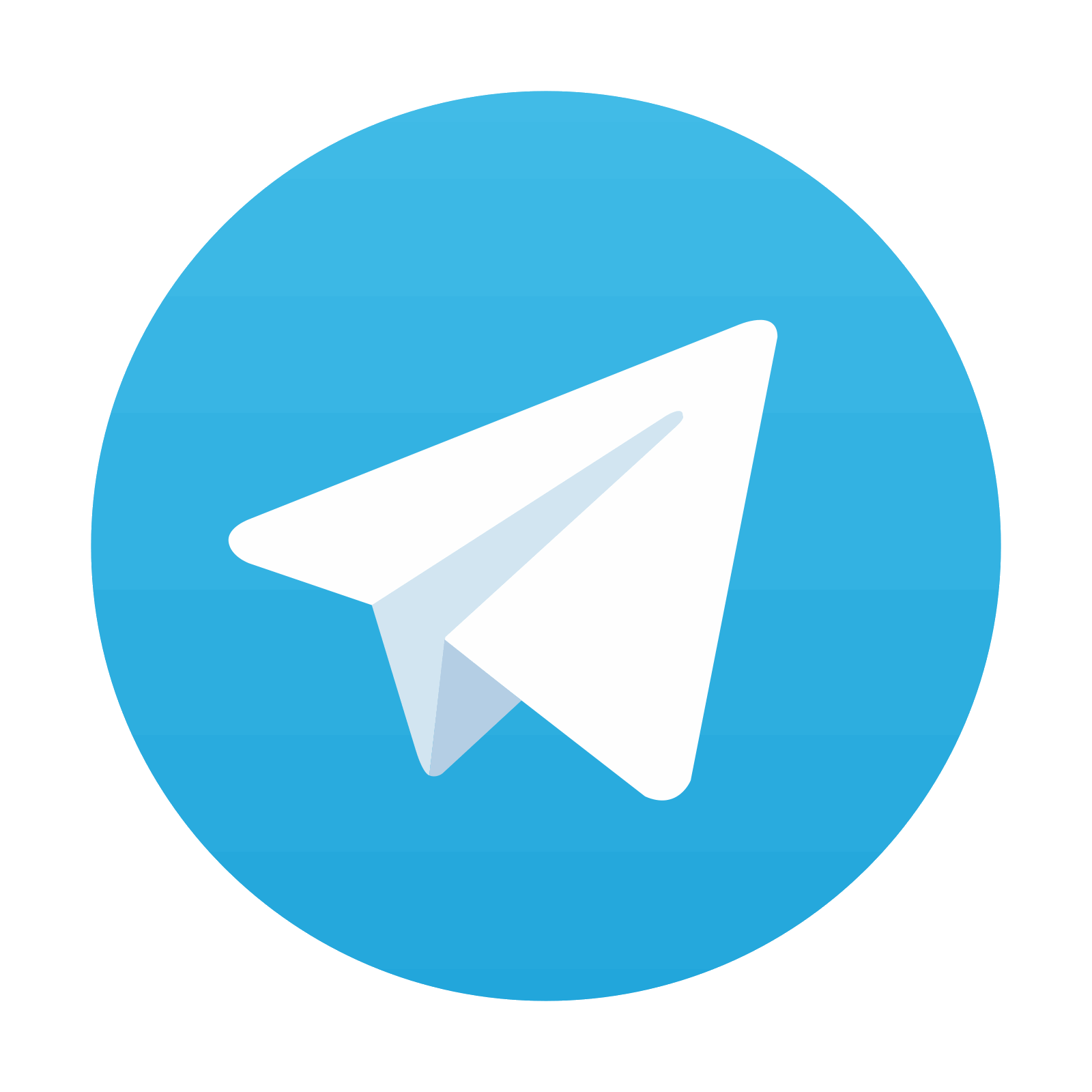
Stay updated, free articles. Join our Telegram channel
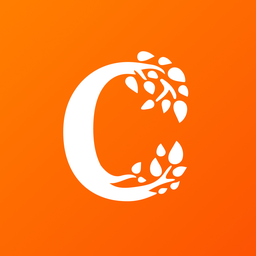
Full access? Get Clinical Tree
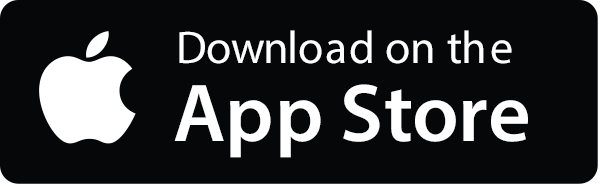
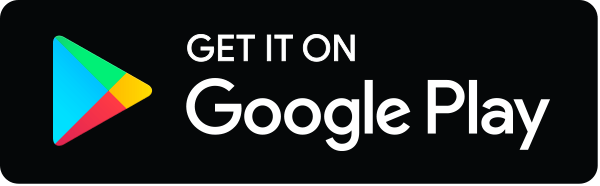