Fig. 13.1
(a) and (b) Illustration of the skeletal anatomy of the thorax. (a) Anterior (front) view. (b) Posterior (back) view (Reproduced with permission from Grant’s Atlas of Anatomy, 13th edition, Lippincott, Williams and Wilkins, 2013)
13.1.1.2 Lungs and Mediastinum
The left lung consists of two lobes, the upper (superior) and lower. The right lung consists of three lobes, the upper, middle and lower lobes (Fig. 13.2). The lungs are covered in a serous membrane called the visceral pleura. The parietal pleura lines the inner surface of the chest wall, covers the diaphragm and encloses the structures in the middle of the thorax. The central region of the thoracic cavity is called the mediastinum (Fig. 13.2). The mediastinum is bordered in front by the sternum and in back by thoracic vertebrae and contains the heart, the great vessels entering and leaving the heart, thymus gland, esophagus and lower portion of the trachea, thoracic duct and thoracic lymph nodes, and nerves passing into and through the thorax, including the vagus and phrenic nerves.
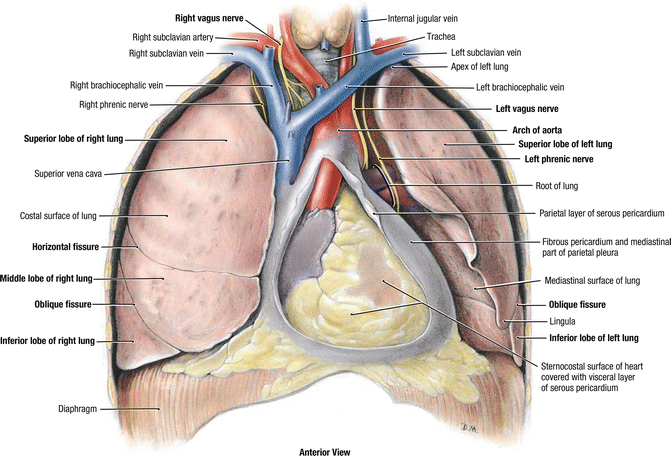
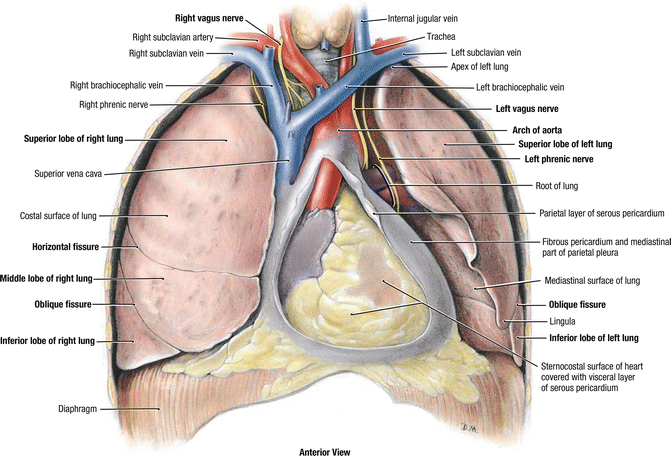
Fig. 13.2
Diagram of the lungs. The right lung has upper, middle and lower lobes. The left lung has upper and lower lobes. The mediastinum, in the central region of the thorax, contains the heart and great vessels and other structures as illustrated (Reproduced with permission from Grant’s Atlas of Anatomy, 13th edition, Lippincott, Williams and Wilkins, 2013)
13.1.1.3 Heart and Great Vessels
The heart (Fig. 13.3) is a hollow muscular organ that lies in the lower part of the thoracic cavity in the middle mediastinum. It is roughly the size of a man’s fist, weighing 300 g in the adult male and 250 g in the female. The heart is divided into four chambers, left and right atria, and left and right ventricles. The right atrium receives the returning deoxygenated blood from all body tissues except the lungs. This returning blood enters the right atrium, through the superior and inferior venae cavae. The right ventricle pumps the deoxygenated blood though four pulmonary veins. From the left atrium, the oxygenated blood goes to the thick- walled left ventricle, which pumps it out to through the aorta to all parts of the body except the lungs.
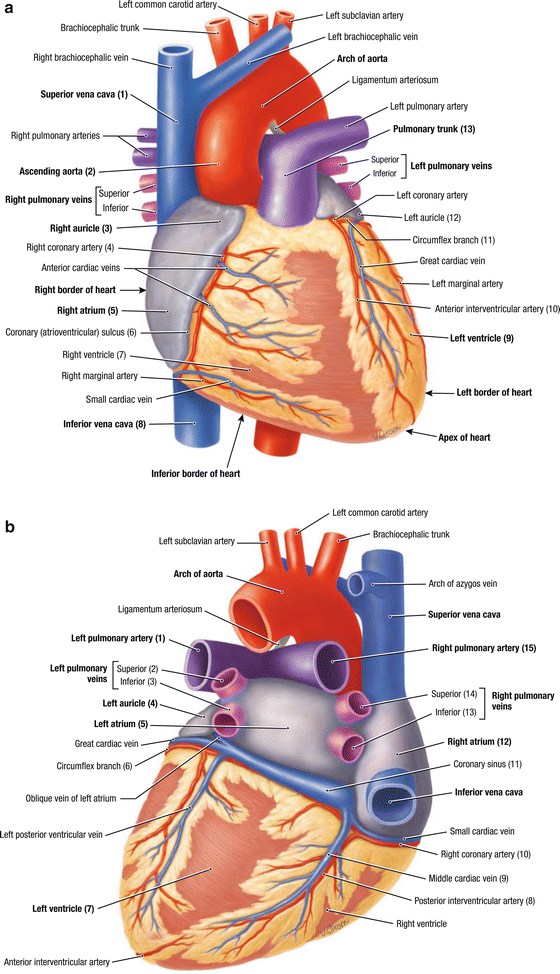
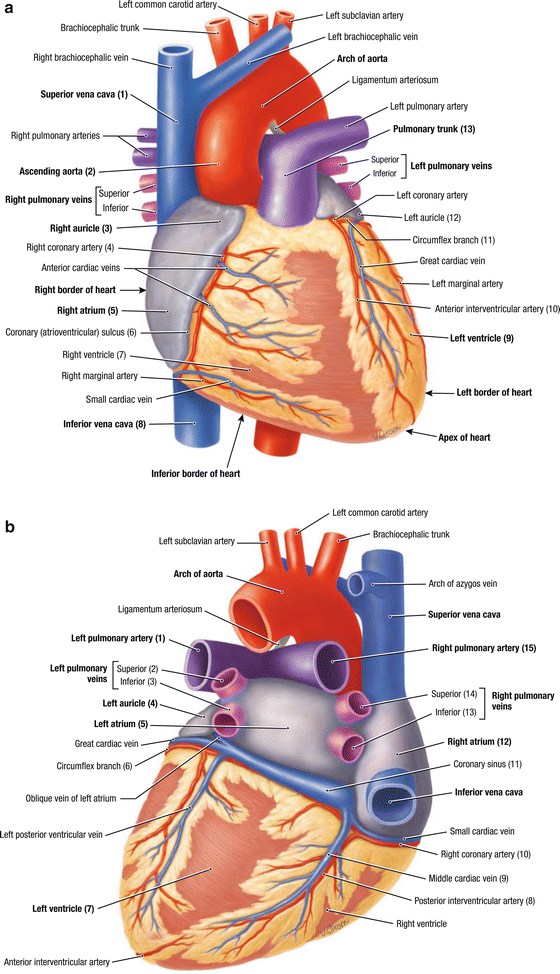
Fig. 13.3
(a) and (b). Diagram of the heart showing right and left atrium, right and left ventricles and great vessels. (a) Anterior view. (b) Posterior view (Reproduced with permission from Grant’s Atlas of Anatomy, 13th edition, Lippincott, Williams and Wilkins, 2013)
13.2 Injury Scaling Methods
13.2.1 AIS
The standard method for classifying the level of injury to a body region or organ is the Abbreviated Injury Scale (AIS) of the Association for the Advancement of Automotive Medicine (AAAM), periodically updated by the Committee on Injury Scaling. The last update was in 2008 [4]. The numerical rating system ranges from 0 (no injury) to 6 (maximum, virtually unsurvivable). The higher the AIS level, the higher the mortality or threat to life. The scale does not quantify long term disability or medical and societal costs of injury. Typical AIS injuries to the rib cage and to thoracic soft tissues are shown in Table 13.1.
Table 13.1
Skeletal and soft tissue injuries to the thorax (Ranked by AIS)
AIS | Skeletal injury | AIS | Soft tissue injury |
---|---|---|---|
1 | 1 rib fracture | 1 | Contusion of bronchus distal to main stem |
2 | 2 rib fractures | 2 | Partial thickness |
Sternum fracture | Bronchus tear distal to main stem | ||
3 | 3 or more fractures | 3 | Lung contusion NFS |
Unilateral flail chest with 3–5 flail ribs | Heart laceration, no perforation | ||
4 | Unilateral flail chest with >5 flail ribs | 4 | Bilateral lung laceration NFS |
Minor aortic laceration Major heart contusion | |||
5 | Bilateral flail chest | 5 | Major aortic laceration |
Tension pneumothorax | |||
6 | 6 | Aortic laceration with hemorrhage | |
not confined to mediastinum |
Regarding rib fractures, one rib fracture is AIS1, two fractures is AIS2 and 3 or more fractures is AIS3. Flail chest can range from AIS3 to AIS5. A flail chest is an unstable chest wall in which a portion of the rib cage does not rise on inspiration because of loss of structural rib cage integrity. Unilateral flail chest is rated AIS 3 if 3–5 ribs are involved and AIS 4 if more than five ribs are involved. Bilateral flail chest is rated AIS 5. In cadaveric studies Walfish et al. considered flail chest as two fractures each of four consecutive ribs [5]. At an international meeting in Paris it was proposed that two fractures of three or more consecutive ribs be defined as flail chest [6]. The Walfisch criterion or nine or more rib fractures to the thorax was considered flail chest in a study by Viano [7].
13.2.2 ISS and PODS
The Injury Severity Scale (ISS) is a measure of the probability of survival. It is obtained by summing the square of the highest AIS in each of three body regions and was developed to account for the effect of injuries to multiple body regions. The ISS uses six body regions: head and neck, face, chest, abdomen and pelvis, extremities and bony pelvis, and external [8]. The Probability of Death Score (PODS) and PODSa (which accounts for subject age) were developed for the same purpose [9]. The equation for PODS is as follows:
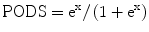
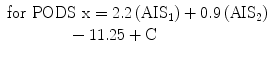
where C = −0.764 for cars, and AIS1 and AIS2 are the first and second highest AIS.
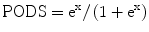
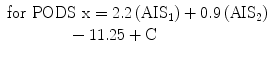
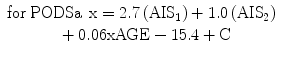
The advantages of PODS over ISS are that PODS has a better goodness–of–fit to real data than ISS and has an inherent definition to health outcome (probability of death) [10]. Whereas AIS, ISS and PODS quantify injury in terms of threat to life, other concepts have been developed to quantify injury in terms of additional factors, such as quality of life or societal cost of injury. These methodologies include HARM and the Injury Priority Rating (IPR). HARM establishes an economic value to each level of injury [11, 12]. Using HARM, chest injury ranked second only to head injury in overall number of fatalities and serious injuries [11]. Hirsch et al. used AIS to rank injuries by impairment [13]. Impairment severity ranged from one to four, with four being most severe. Six different aspects of impairment (mobility, cognitive/psychological, cosmetic, sensory, pain, and daily living) for three different durations (0-1 year, 1-5 years, > 5 years) were thus ranked. Carsten and O’Day developed the Injury Priority Rating (IPR) to evaluate overall impairment [14]. Carsten modified this and developed the Multi-Injury Priority Rating (MIPR) to express overall injury impairment [15]. The IPR provides for post-accident survival and can distinguish the impairment due to injuries with the same AIS value. Marcus extended impairment studies to more recent data and reviewed IPR and impairment methodologies [16].
13.3 Thoracic Injury and Mechanisms
13.3.1 General
In earlier work, Cohen reported that for the thorax and abdomen, most of the contact for the driver was to the steering assembly, while for the passenger, it was the instrument panel [17]. The NASS data base from 1979 to 1984 was reviewed by Haffner for passenger cars in non-ejection and non-rollover cases and published by Schneider et al. [18]. For drivers and passengers, skeletal injury represented the highest percentage of thoracic AIS, followed by pulmonary/lung injuries for the driver and then liver and heart injuries. The liver and spleen are located under the diaphragm, but are bounded by the lower lateral rib cage. The rim of the steering wheel or the instrument panel can produce soft tissue injuries to these components. Although arterial injuries accounted for 6–8 % of AIS >2, they accounted for 27–30 % of estimated HARM. With the increased use of seat belts and the requirement for frontal air bags, these injury statistics have changed. More current data are reviewed in subsequent sections.
13.3.2 Rib Fractures and Flail Chest
Rib fracture and flail chest occur with blunt impact to the rib cage. Rib fractures are the most frequent type of AIS3+ chest injuries [19]. It is probable that ribs fail in bending, with failure occurring on the tensile side of the ribs. Stalnaker and Mohan, and Melvin et al. concluded that maximum compression of the chest is the determining factor for rib fracture [20, 21]. In their review of injury and response data from cadaveric studies, rib fractures were more frequent with chest deflections over 3 in., while none occurred at deflections less than 2.3 in. Thus, it appears that the number of rib fractures depends on the magnitude of rib deflection, rather than the rate of deflection. However, the amount of force depends on the rate at which the force is applied due to the viscous nature of the thorax. Thus, for a given loading rate, force appears to be related to the number of rib fractures. Eppinger developed a relationship between the number of rib fractures and upper torso shoulder belt force, age and weight of cadaveric subject [22].
In 2008, Trosseille et al. showed a promising methodology to examine rib fracture mechanisms, using strain gauges glued on the ribs of PMHS [23]. In 2009, they published the results of static airbag tests performed on 50th percentile male PMHS at different distances and angles (pure lateral and 30° forward oblique direction) [24]. To complete the study, Leport et al. carried out eight PMHS lateral and oblique impactor tests with the same methodology [19]. The rib cages were instrumented with more than 100 strain gauges on the ribs, cartilage and sternum. A 23.4-kg impactor was propelled at 4.3 or 6.7 m/s. The forces applied to the PMHS at 4.3 m/s ranged from 1.6 to 1.9 kN and the injuries varied from 4 to 13 rib fractures.
Kindig et al. [25] identified rib-level differences in fracture characteristics for individual ribs subjected to anterior-posterior loading. Twenty-seven individual ribs from levels 2 to 10 from 3 postmortem human subjects (two females and one male) were tested in anterior-posterior loading at a quasistatic (2 mm/s) loading rate. Rib 2 was 3–4 times stiffer than rib 3, whereas all other ribs were comparable in stiffness to rib 3. Fracture forces, fracture displacement, and work to fracture showed no clear rib-level differences. The data were compared to a similar study at dynamic loading rates (1.43–1.85 m/s). The quasistatic tests exhibited lower peak force and greater normalized fracture displacement than the dynamic tests, though the work was comparable between the 2 studies [25].
13.3.3 Lung Contusions
Unlike rib fractures, lung contusion is rate dependent. Fung and Yen determined that it was a velocity dependent phenomenon [26]. At high velocities, a compression or pressure wave is transmitted through the chest wall to the lung tissue, causing damage to the capillary bed of the alveoli. Lacerations of lung tissue can also occur at sites of rib fractures. Gayzik et al. induced pulmonary contusion in male Sprague Dawley rats (n = 24) through direct impact to the right lung at 5.0 m/s [27]. Force vs. deflection data were collected and used for model validation and optimization. CT scans were taken at 24 h, 48 h, 1 week, and 1 month post contusion and used to quantify the volume of pathologic lung tissue. The best fit injury measure at the 24-h time point was the product of maximum principal strain and strain rate, with a value of 28.5 s−1. The maximum principal strain and the maximum principal strain rate also characterized pathology well, with the threshold for lung injury at 24 h post-impact being 15.4 % and 304 s−1, respectively.
13.3.4 Hemothorax and Pneumothorax
Hemothorax is bleeding into the pleural space. Blood vessels can be lacerated by broken ribs, leading to hemothoax. If a hole is created in the pleural sac between the lungs and the rib cage, a pneumothorax occurs. The puncture or laceration can be in the parietal pleura lining the inside of the rib cage or in the visceral pleura surrounding the lungs. The pneumothorax is often the result of rib fracture. Thus, rib fracture, hemothorax and pneumothorax appear to be deflection dependent, while lung contusion appears to be velocity dependent.
13.3.5 Heart Injuries
During high-speed thoracic impact the heart can be subject to contusion, laceration or cardiac arrest. Lasky et al. in a review of 67 cases of frontal and side impact collisions by the Vehicle Trauma Research Group at UCLA found that in frontal collisions, energy absorbing steering wheel hubs and columns resulted in reduced cardiovascular injury [28]. Side impact collisions were shown to produce a large proportion of the cardiovascular injury. Contusion appears to be due to compression and the velocity of compression while cardiac laceration may be due to high magnitudes of compression over the sternum. At high rates of loading, the heart may undergo fibrillation or arrest. High speed blunt impacts (15–20 m/s) appear to interrupt the electromechanical transduction of the heart wall. Cooper et al. reported on 38 mid-sternal impacts to the chest of pigs by a cylindrical mass of 0.14–0.38 kg and 3.7 and 10 cm in diameter at velocities of 20–74 m/s [29]. Acute ventricular fibrillation appeared to be associated with mid-sternal blows during the T-wave of the electrocardiogram (ECG). Kroell et al. reported 11 cases of ventricular fibrillation (VFB) in impacts to the mid-sternum of 23 anesthetized swine [30]. Eight of the VFB’s were immediate and five of these were in impacts which occurred during the T-wave of the ECG.
13.4 Effects of Age on Injury Tolerance
Zhou et al. studied the effects of age on thoracic injury tolerance by analyzing the mechanical properties of human bones and soft tissues and examining results from literature on thoracic impact tests to PMHS [31]. The work divided the age range into three groups: 16–35, 36–65, and 66–85 years. The reduction ratios with age for thoracic injury tolerance of AIS 3 ranged from 1 to 0.47 to 0.28 for belt loading, for these three age groups; the tolerance decreased from 1.0 to 0.84 to 0.79 for blunt frontal impacts and from 1 to 0.80 to 0.73 for side impact loads. Tolerance under concentrated loading such as seat belts appeared to be governed more by the properties of the rib cage and tolerance under blunt loading appeared to be governed mainly by the properties soft tissues. In computer simulations by Kent et al. the structural and material changes played approximately equal roles in the force–deflection response of the thorax [32]. Changing the rib angle to be more perpendicular to the spine as in the older person increased the effective thoracic stiffness, while the “old” material properties and the thin cortical shell decreased the effective stiffness. All three effects tended to decrease chest deflection tolerance for rib fractures. The findings indicated an older person’s thorax, relative to a younger, does not necessarily deform more in response to an applied force but the tolerable sternal deflection level is much less.
Mertz and Dalmatos assessed the effectiveness of 3-point restraint systems using 1988–2005 NASS data for 3-point belted, front outboard-seated, adult occupants in passenger vehicles equipped with airbags and involved in frontal, tow away collisions [33]. These data showed that (i) half of the occupants with AIS ≥ 3 chest injuries were in collisions with a ΔV ≤ 40 km/h; (ii) for older occupants (50+ years), half experienced their chest injuries at ΔV ≤34 km/h; and (iii) the chest injury rate for the older occupants was more than double that of the younger occupants. Analysis indicated that 2.5 kN shoulder belt limit load would substantially reduce shoulder belt-induced AIS ≥ 3 chest injuries in 99 % of frontal collisions to all adult, front outboard seated occupants whose normalized bone strengths were greater than 0.4.
Cormier [34] assessed the influence of body mass index (BMI) on thoracic injury potential. The data for this study were obtained from the National Automotive Sampling System-Crashworthiness Data System (NASS-CDS) database for years 1993–2005. Obese occupants had a 26 % and 33 % higher risk of AIS 2+ and AIS 3+ thoracic injury when compared to lean occupants. The increased risk of AIS 3+ injury due to obesity was slightly higher for older occupants, but the influence of age was greater than that of obesity. The increase in injury potential was higher for unbelted obese occupants than unbelted. Non-parametric and parametric risk curves were developed to estimate the risk of thoracic injury based on occupant BMI, belt use and delta-V. Overall, increase in thoracic injury risk due to obesity was more prominent in males and older occupants and for occupants sustaining AIS 3+ thoracic injuries [34].
Koppel et al. [35] studied data from the Australian Transport Accident Commission insurance claims database for two groups of drivers: aged 41–55 years (middle-aged drivers) and aged 65 years and older (older drivers). The majority of crashes involved a collision with another vehicle (70.0 % of middle-aged drivers and 68.7 % of older drivers). Older drivers sustained a significantly higher proportion of injuries to the thorax (30.9 % compared to 18.5 % of middle-aged drivers). Conversely, a significantly higher proportion of middle-aged drivers sustained injury to the neck (30.6 % compared to 12.1 % of older drivers) [35].
13.5 Aortic Trauma
13.5.1 Epidemiology
Previous studies showed that traumatic rupture of the aorta (TRA) accounted for 10–25 % of all deaths from MVCs [36–39]. Previous studies estimated that 7,500–8,000 cases of blunt aortic injury occurred annually in the US and Canada [40, 41]. Eighty to eighty-five percent of the victims of TRA died at the scene of the accident [42]. In a prospective study using 50 trauma centers in North America Fabian et al. reported 274 (199 men, 75 women, mean age 38.7 years) blunt aortic injury cases studied over 2.5 years, of which 81 % were caused by automobile crashes [43]. Overall mortality was 31 %, with 63 % of deaths attributable to the aortic rupture itself. Motor vehicle crashes were responsible for 222 of the 274 cases with 72 % of these being head-on and 24 % from side impacts. The other causes of TRA were motorcycle crashes, auto-pedestrian impacts, falls and other. Forty-six % had multiple rib fractures, 38 % pulmonary contusion, 31 % pelvic injury and 51 % closed head injury. In a study of 63,763 patients with blunt trauma over a 5-year period, 8.1 % sustained pelvic fractures and 441 (1.6 %) had aortic ruptures. Eighty-six had both aortic rupture and pelvic fracture. The incidence of aortic rupture in the pelvic fracture cases was more than twice that in the overall blunt trauma population. Mechanisms of injury were predominantly high-speed motor vehicle accidents followed by falls and being struck as a pedestrian [38].
Bertrand et al. [44] analyzed crash data collected from 1998–2006 as part of the Co-operative Crash Injury Study (CCIS) to assess frontal, near-side, and far-side injury risks related to TRA. The database included 15,074 occupants with detailed autopsy reports. The influences of gender, age, Equivalent Test Speed (ETS), compartment intrusion, and restraint system on TRA were analyzed. TRA occurred in 1.2 % of all occupants but accounted for 21.4 % of all fatalities. The incidence of TRA was higher in side impacts (2.4 %) than in frontal impacts (1.1 %). TRA injury risk increased with ETS, intrusion, and age and decreased with the absence of intrusion regardless of the impact direction. It also decreased for belted occupants in frontal impacts. Multiple rib fractures were the most common injuries associated with TRA (79.1 %). TRA victims with uninjured or slightly injured (AIS 1) rib cage were significantly younger (p < 0.0001) than other TRA victims. Whatever the impact type, the TRA victims sustained mostly bilateral rib fractures. Fractures concerned mainly the 2nd up to the 7th ribs of TRA victims. The typical TRA involving partial or complete aorta transection within the peri-isthmic region was independent of age and impact type. The high frequency of bilateral rib cage fractures observed in TRA victims and the significant influence of intrusion on TRA occurrence emphasized that the aortic injury mechanism mainly involves a severe direct chest impact or compression [44].
13.5.2 Anatomical Location of Aortic Injury
Sites of aortic laceration include the aortic isthmus, root, and aortic insertion into the diaphragm. Ninety percent of thoracic aortic injuries occur in the region of the aortic isthmus, just distal to the origin of the left subclavian artery [45]. The isthmus is a portion of the aorta between the ligamentum-arteriosum and left subclavian artery (Fig. 13.3) that develops from the fetal ductus arteriosus. The aortic isthmus averages only about 1.5 cm in length in adults and the region of the proximal descending aorta is often referred to loosely as the region of the aortic isthmus [45]. Clinically, thoracic aortic injury involves the ascending aorta in only 5 % of cases. At autopsy, it was the site of injury in 20–25 % of cases. This area of the aorta is associated with grave complications such as valve rapture and coronary artery laceration that are threats to life.
13.5.3 Aortic Injury Mechanisms
Three general mechanisms for TRA have been proposed: (1) traction or shear forces generated between relatively mobile portions of the vessel and points of fixation, (2) direct compression over the vertebral column, (3) and excessive sudden increases in intraluminal pressure [45]. Hossack et al. in an Australian study pointed out that 12.7 % of fatal road crash victims had a rupture of the aorta [46]. He also noted that the recurrent laryngeal nerve hooks around the aorta just near the ligamentum-arteriosum and that forcible traction on this nerve may exert pressure at the site of aortic tears. Viano [47] noted that inertial loading of the blood-filled heart can cause the heart to displace in the chest cavity and stretch points of attachment of the aortic arch such as the superior arteries or the ligamentum-arteriosum [47]. This may occur if the heart is displaced vertically, laterally or obliquely. Aortic lacerations generally have a transverse orientation. Mohan and Melvin showed that the descending mid-thoracic aorta failed in the transverse rather than longitudinal direction under uniform biaxial stretch [48]. Lasky et al. postulated that large fluid pressures resulting in a water hammer effect may be important in causing vessel rupture [28].
Newman and Rastogi observed that in all 12 cases of aortic rupture, the impact was not directly frontal, suggesting that a transverse component of impact is required to produce aortic wall laceration between fixed and mobile sections of the aorta [49]. In cadaveric aide impact sled tests performed by Cavanaugh et al. the impacts were to the left side of the PMHS at 6.7 and 9 m/s with one test at 10.4 m/s [50, 51]. In these tests, the sudden deceleration of the sled caused the unembalmed PMHS to slide across a TeflonTM seat into a side wall instrumented with load cells. The arterial system of the torso was pressurized to 2 psi and the venous system to 1 psi. After the impact, each PMHS had a necropsy performed by a board-certified pathologist. In five of 17 tests, a tear of the aortic arch was noted. All tears were in the transverse direction. The authors hypothesized that the more mobile heart and aortic arch translated laterally, producing tears at the top of the more firmly anchored descending aorta.
Shah et al. determined mechanical properties of planar aorta tissue at high strain rates [52]. Cruciate tissue samples from various regions of 12 PMHS thoracic aortas were subjected to equi-biaxial stretch at two nominal speed levels using a new biaxial tissue-testing device. The aorta tissue exhibited nonlinear behavior. In a series of component-level tests, the response of the intact thoracic aorta to longitudinal stretch was obtained using seven aorta specimens. The aorta failed within the peri-isthmic region with transverse tears and the intima failed before the media or adventitia. Complete transection was observed at 92 N axial load and 0.221 axial strain.
Hardy et al. investigated TRA mechanisms in PMHS in four quasi-static and one dynamic tests [53]. The quasi-static tests included anterior, superior, and lateral displacement of the heart and aortic arch in the mediastinum, resulting in partial tears to complete transection. All injuries occurred within the peri-isthmic region. The average failure load and stretch were 148 N and 30 %, respectively, for the quasi-static tests. The results indicated that intraluminal pressure and whole-body acceleration are not required for TRA to occur and that the role of the ligamentum-arteriosum is likely limited. The studies indicated that tethering of the descending thoracic aorta by the parietal pleura was a principal aspect of this injury.
Hardy et al. investigated the mechanisms of TRA in eight unembalmed PMHS which were inverted and tested in various dynamic blunt loading modes [54]. Impacts were conducted using a 32-kg impactor with a 152-mm face. High-speed biplane x-rays of radiopaque markers on the aorta were used to visualize aortic motion. Clinically relevant TRA was observed in seven of the tests. Peak average longitudinal Lagrangian strain was 0.644 and the average peak strain for all tests was 0.208 +/− 0.216. Peak intraluminal pressure was 165 kPa. Longitudinal stretch of the aorta was found to be a principal component of injury causation. Stretch of the aorta was generated by thoracic deformation, which was required for injury to occur. Atherosclerosis further promoted injury.
13.6 Biomechanics of Frontal Impact
Many biomechanical tests have been performed on PMHS under controlled laboratory conditions to measure biomechanical responses (forces, accelerations, deformations, pressures) and obtain details of resulting injury through necropsy of the body after impact. Pendulum and sled tests have been performed to ascertain these data for frontal and lateral impacts. This data has been used to develop frontal and side impact dummies and to develop injury criteria. A description of these tests follows.
13.6.1 Biomechanical Response of the Thorax in Frontal Impact
13.6.1.1 Pendulum Impacts to the Sternum
Some of the most extensive testing analyzed in the 1970s involved six-inch diameter rigid pendulum impacts to the sternum of unembalmed PMHS (Fig. 13.4). The data were presented by Kroell et al., Nahum et al., and Stalnaker et al. [55–60]. These data have also been analyzed by Lobdell et al. and Neathery [61, 62]. In the Kroell tests, a six-inch diameter impactor contacted the sternum at the level of the interspace between the fourth and fifth ribs. Figure 13.5 shows force- deflection curves for 4.02–5.23 and 6.71–7.38 m/s in the Kroell et al. tests.
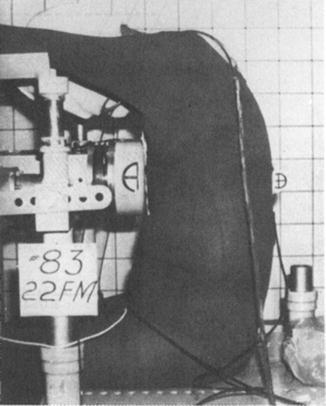
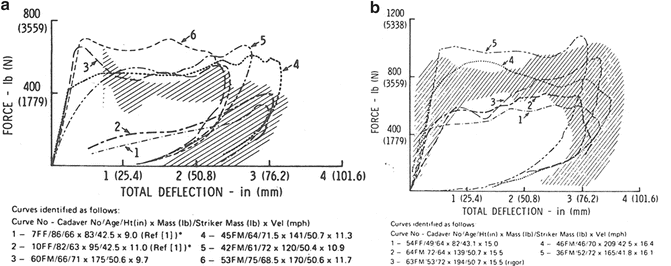
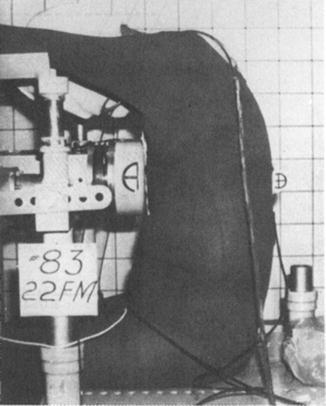
Fig. 13.4
Photograph showing a frontal pendulum impact applied to the sternum (From [55], reproduced by permission of the Stapp Association)
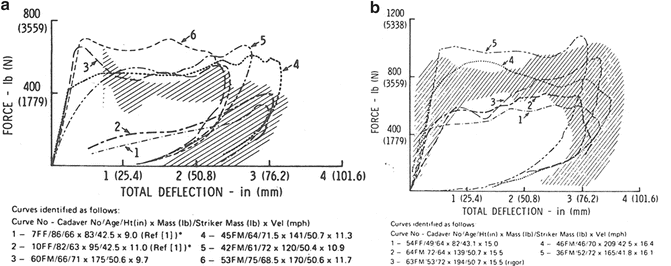
Fig. 13.5
(a) and (b) Force-deflection plots generated by Kroell et al. [56] for frontal impacts to the sternum. (a) 4.02–5.23 m/s impacts. Shaded area represents a corridor of three tests at 5.14 m/s with 19.3 kg mass. (b) 6.71–7.38 m/s impacts. Shaded area represents a corridor of seven tests at 6.7-7.4 m/s with 23.1 kg mass. The impactor had a 6 in. diameter flat, rigid surface and a mass of 19.3 or 23.1 kg (Reproduced by permission of the Stapp Association)
The total chest deflection including the flesh overlying the sternum was included by Kroell et al. [55, 56]. Neathery developed corridors of skeletal chest deflection, based on these data [62]. Lobdell et al. showed that lower impactor masses resulted in lower deflections [61]. Patrick developed force-deflection curves under conditions similar to the Kroell unrestrained back conditions, but used himself as a volunteer and a padded (2.4 cm Rubatex R310V) six-inch diameter striker at velocities of 2.4–4.6 m/s [63]. Forces in the tensed condition were slightly greater than in the relaxed condition for the same impact velocity. Apparent initial stiffness were: 79 N/mm at 2.4 m/s for tensed, 57 N/mm at 2.4 m/s for relaxed and 250 N/mm at 4.6 m/s for tensed conditions. Peak skeletal deflections were 44–46 mm (16–17 % deflection of the rib cage).
The idealized force-deflection curves derived from these responses can be divided into a loading and an unloading phase (Fig. 13.6), with the loading phase having three components [64]. An initial rapid rise or apparent initial stiffness (A) is due in large part to the viscous properties of the thorax. The force plateau (B) is also due to a viscous response. At maximum deflection (C), the impactor and subject are moving at a common velocity and the forces are due to inertial forces caused by whole-body acceleration and the elastic forces due to tissue compression. The unloading portion of the curve (D), is due to unloading of the compressed tissues, and follows the elastic non-linear unloading of the thorax seen in quasi-static tests.
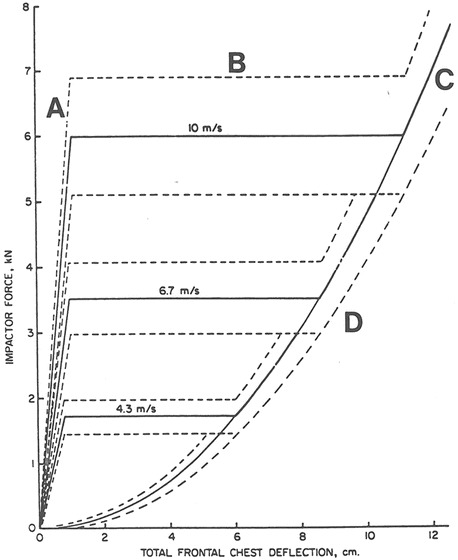
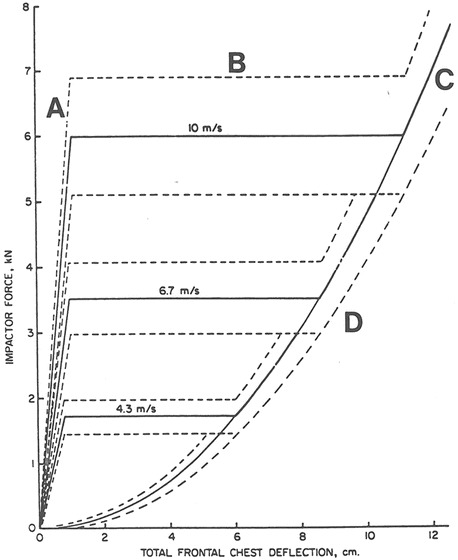
Fig. 13.6
Plots of pendulum impact force-deflection response of the thorax illustrating the various components of the curve. A. Rise. B. Plateau. C. Maximum deflection D. Unloading. See text for equations used to generate various portions of these plots. Based on pendulum impacts using 6 in. diameter rigid flat disc and 23.4 kg impact mass [162]
13.6.1.2 Belt Loading
Beginning in the early 1960s, three-point restraint systems have been installed in some vehicles. However, in the 1987 model year all vehicle manufacturers had to certify that their cars would meet the 50th percentile, adult male protection requirements in the 48 km/h frontal, rigid-barrier test specified in FMVSS 208. Manufacturers began installing 3-point restraints with force-limiting shoulder belts and frontal airbags for the driver and right front passenger [33].
Fayon et al. calculated the resultant normal force to the thorax from the geometry and tension forces at belt anchorages and determined that sternal thoracic stiffness was 166.4 N/mm [65]. Walfisch came up with slightly smaller values of 70–116 N/mm (mean 119.4 N/mm) [5]. L’Abbe et al. conducted static and dynamic tests and belt loading and consequent chest deflection in human volunteers [66]. The dynamic loads were up to 3,600 N and produced mid-sternal stiffness of 137 N/mm, right 7th rib stiffness of 123 N/mm and 200 N/mm at left clavicle. The Hybrid III dummy tended to produce stiffer responses than relaxed volunteers, with better agreement to tensed volunteers, particularly at mid-sternum. Backaitis and St.-Laurent in similar tests showed greatest deflections at the seventh rib in volunteers and least in the shoulder, while Hybrid III showed maximum deflections at the shoulder [67].
13.6.1.3 Two-Point Belt Loading Plus Knee Bolster
Cheng et al. performed eleven PMHS tests with a VW two- point belt and knee bolster in the right-front passenger position [68]. The belt crossed from the right shoulder to the left side. There were more rib fractures on the left in seven cadavers. The number of rib fractures ranged from 0 to 14, with seven subjects having seven or more rib fractures. Peak upper shoulder belt load was 5.1–7.6 kN for 48 km/h impacts with peak sled deceleration of 22 g. The peak belt loads ranged from 3.6 to 9.7 kN for four runs with peak sled deceleration of 35 g. The ratio of lower to upper belt peak loads was approximately 0.9.
Kent et al. tested 15 PMHS in single and double diagonal belts and distributed, and hub loading on the anterior thorax [69]. Subjects were positioned supine on a table and a hydraulic master–slave cylinder with a high-speed materials testing machine provided controlled chest deflection at rates similar to sled tests. Non-injurious tests were followed by a final injurious test (40 % chest deflection). The distributed loading condition generated the stiffest response (3.33 kN at 4.6 cm), followed by the double diagonal belt condition (3.18 kN at 4.6 cm), single diagonal belt (2.28 kN at 4.6 cm) and hub (1.14 kN at 4.6 cm). Corridors were developed to a deflection level of 20 % of the 50th percentile male’s external chest depth. Kindig et al. studied of force-displacement and kinematic responses under a highly-localized loading condition using three PMHS (ages 44, 61, and 63 years) ribcages [25]. In general, bilateral loading produced an approximately symmetric deformation pattern, while unilateral loading resulted in approximately twice the resultant deformation on the ipsilateral side compared to the contralateral side.
Forman et al. [70] investigated a 3-point restraint system in the rear seat with a force-limiting (FL) and pretensioning (PT) shoulder belt in a series of 48 km/h frontal impact sled tests (20 g, 80 ms sled acceleration pulse) performed with PMHS [70]. The results of these tests were compared to matched PMHS tests, published in 2008, performed in the same environment with a standard 3-point belt restraint. The FL + PT restraint system resulted in significant (p < 0.05) decreases in peak shoulder belt tension (average ± standard deviation: 4.4 ± 0.13 kN with the FL + PT belt, 7.8 ± 0. 6 kN with the standard belt) and 3 ms-resultant, mid-spine acceleration (FL + PT: 34 ± 3.8 g; standard belt: 44 ± 1.4 g). The FL + PT tests also produced more forward torso rotation caused by decreased forward excursion of the pelvis and increased payout out of the shoulder belt by the force-limiter.
13.6.1.4 Quasi-Static Tests
As three point belts and air bags are more frequently used, lower rate loading will become more important in frontal impact. The distributed loading to the ribs due to the airbag, and rib and clavicle loading due to the shoulder belt make the biomechanical response of the ribs and clavicle (in addition to the sternum) increasingly important. Thus, in addition to the dynamic pendulum data from sternal impacts, quasi-static chest loading data is also important. These data are described below.
Stalnaker et al. loaded the sternum of volunteers and PMHS with a 153-mm diameter rigid plate with the subject’s back against a rigid wall [60]. Stiffness averaged 12.2 N/mm for unembalmed PMHS, 40.2 N/mm for relaxed and 114 N/mm tensed volunteers. Lobdell et al. showed much less stiffness under similar loading conditions: 7 N/mm for relaxed volunteers and 23.7 N/mm for the tensed subject [61].
Tsitlik et al. [71] measured force versus deflection due to loading the sternum of eleven patients with a 48 × 64 mm rubber thumper [71]. The average stiffness was 9.1 N/mm (range: 5.25–15.9 N/mm) for deflections of 30–61 mm. At WSU, Cavanaugh et al. loaded the sternum and rib cage of three unembalmed PMHS with 25-mm strokes at quasi-static loading rates (described in [72]). The thorax stiffness were as follows: upper and mid-sternum, 8.6–12.3 N/mm; lower sternum, 5.7–11.4 N/mm; 2nd rib, 5.6–7.3 N/mm; 5th rib, 5.1–8.4 N/mm and 7th rib, 3.4–5.2 N/mm. Weisfeldt loaded the chest of volunteers at 60 Hz with a 22 × 64 mm pad [73]. The mean stiffness was 6.3 N/mm. On one subject loading the chest with a 153-mm diameter pad resulted in a stiffness of 21 N/mm. Melvin et al. after reviewing the quasi–static load–deflection literature, concluded that up to deflections of 41 mm the thorax has an approximate linear stiffness of 26.2 N/mm and for deflections greater than 76 mm, the linear stiffness is 120 N/mm [74]. Melvin also modeled these force–deflection relationships with a quadratic equation:
where K = 47.6 N/mm2.

Fayon et al. demonstrated stiffness of 17.5–26.3 N/mm due sternal deflections up to 25 mm for static belt loading to supine volunteers [65]. This compared to 8.8–17.5 N/mm for disc loading up to 38 mm. At the 2nd and 9th ribs the estimated stiffness were 17.5–35 N/mm and 8.8–17.5 N/mm. Melvin and Weber estimated an apparent stiffness from the static belt tests of L’Abbe et al. [64, 66] (1982). These results were 67.5 N/mm at mid-sternum, 40 N/mm at right 7th rib, and 95 N/mm at left clavicle for deflections up to 10 mm and estimated normal forces up to 667 N. Melvin has suggested that these data may be significantly higher than that of Fayon et al. because spinal curvature may have reduced stiffness in the latter study. Arbogast et al. compared thoracic force-deflections measured during adult cardiopulmonary resuscitation (CPR) to that measured during hub-based loading of adult PMHS [75]. A load cell and accelerometer was integrated into a monitor-defibrillator to measure chest compression and applied force during live human CPR. Chest response during CPR demonstrated more hysteresis than the PMHS, indicating the viscoelastic nature of the thorax is more pronounced when tissues are naturally perfused and substantial tissue autolysis has not begun.
13.6.2 Injury Tolerance of the Thorax in Frontal Impact
Much of the early work on frontal impact tolerance was reviewed by Mertz and Kroell [76]. Some of this data and later data are summarized below. These data have been used in the development of the Hybrid III frontal impact dummy [77].
13.6.2.1 Acceleration Criteria
Human tolerance for severe chest injury is often stated as the peak spinal acceleration (sustained for 3 ms or longer) not to exceed 60 g in a frontal crash. The Hybrid III dummy is used for assessment of frontal impact crashworthiness per Federal Motor Vehicle Safety Standard 208 (Code of Federal Regulations, Title 49, Part 571.208, 1986). Early acceleration tolerance data was obtained by Stapp, who demonstrated the human tolerance to rocket sled acceleration when belt restraints were worn [78, 79]. Thoracic accelerations up to 40 g for 100 ms or less were tolerated. In one subject a maximum of 45 g was tolerated which resulted in a calculated pressure of 252 kPa under the harness. Peaks of 30 G reached at a rate of 1,000 g/s were not tolerated. Eiband in an analysis of the Stapp data showed that the acceleration tolerance decreased as duration of exposure increased [80]. Spinal acceleration is a general indicator of the overall severity of whole body impact, but is sensitive to changing impact conditions [81]. Mertz and Gadd studied the tolerance and response of a 40 year old male stunt man who took 16 dives from heights of 27–57 ft onto a thick mattress [82]. For each dive the stunt man executed three quarters of a turn and landed supine on the mattress. In ten dives chest acceleration was measured. The authors concluded that 50 g chest acceleration for a pulse less than 100 ms was within voluntary range of healthy adult males. A 60-g acceleration with duration less than 100 ms was recommended as the tolerance level until other the availability of data. This finding was used in the development of the FMVSS 208 chest injury criterion.
13.6.2.2 Force Criteria
Bierman et al. tested young male volunteers with a drop-weight device that loaded a lap/double-shoulder belt harness (490 cm2 in area) [83]. Painful reactions and minor injury occurred when loads exceeded 8.9 kN. When load area was increased to 1,006 cm2, loads of 8.0–13.3 kN were sustained without injury. Patrick et al. performed a series of sled tests with embalmed PMHS to simulate the response of an unrestrained occupant [84]. The head, chest and knees impacted padded load cells. These studies were used as the basis for the design of the energy absorbing steering column. Gadd and Patrick, and Patrick et al. (1969) used a prototype energy absorbing steering column in unrestrained cadaveric sled tests [85]. A 3.3 kN hub load to the sternum and 8.8 kN distributed load to the shoulders and chest resulted in only minor trauma in centered impacts. The reactive force is due to the inertial, elastic and viscous components of the torso.
13.6.2.3 Energy Criterion
Eppinger and Marcus examined the results of 82 laboratory impact tests and concluded that severity of injury to the thorax was proportional to the amount of specific energy that the thorax must absorb [86]. The severity of injury was found to be inversely proportional to the impacted area and the duration of time over which the energy was transferred.
13.6.2.4 Compression Criteria
Kroell et al. analyzed a large number of blunt thoracic impact experiments (Fig. 13.4) and determined that chest compression correlated well with AIS (r = 0.730) while maximum plateau force did not (r = 0.524) [55, 56]. The linear equation relating AIS to compression was:
where C was chest deformation divided by chest depth, resulting in C = 0.3 (30 %) for AIS 2 and 0.4 (40 %) for AIS 4. Thus for the 230 mm chest depth of the 50th percentile male, 30 % compression or 69 mm deflection predicts AIS 2 and 40 % compression or 92 mm deflection predicts AIS 4.

The integral of spinal acceleration which is a measure of the velocity of deformation correlated almost as well as compression to injury severity [59]. In 5–7 m/s sternal impacts to PMHS, compressions > 20 % regularly produced rib fractures. Compressions of 40 % produced flail chest. Neathery et al. extrapolated the 40 % tolerance for severe injury to the Hybrid III frontal impact dummy to obtain maximum allowable compression of 75 mm [62]. Viano demonstrated that severe injury to internal organs occurred at an average maximum compression (Cmax) of 40 % and recommended Cmax of 32 % to maintain enough rib cage stability to protect internal organs [87]. The Federal Motor Vehicle Standards 208 (Code of Federal Regulations, 571.208), allowed a maximum 76 mm chest deflection in the 50th percentile Hybrid III dummy in frontal impact crashworthiness testing of automobiles. This was reduced to 63 mm in the FMVSS 208 standard upgrade, commencing in model year 2003, and including the entire vehicle fleet by model year 2006. The upgrade also included the 5th percentile Hybrid III dummy with a maximum allowable chest deflection of 52 mm [88].
13.6.2.5 Viscous Criterion
Soft tissue injury is compression and rate dependent [81]. Lau and Viano in impact studies over the liver of anesthetized rabbits, found that when maximum compression (Cmax) was held to 16 %, liver injury increased as impact velocity increased from 5 to 20 m/s [89]. In frontal thoracic impacts to anesthetized rabbits at 5, 10 and 18 m/s, severity of lung injury was found to increase with Cmax at each level of velocity [90]. The alveolar region was more sensitive to the rate of loading than regions of vascular junctions. Data from 123 frontal impacts to anesthetized rabbits were used to define the viscous tolerance [91]. This lead to the development of the viscous criterion: VCmax, the maximum product of velocity of deformation and compression is an effective predictor of injury risk, and is a measure of the energy dissipated by the viscous elements of the thorax [92].
Kroell et al. verified the validity of the viscous criterion in blunt thoracic frontal impacts to anesthetized swine [30, 93]. In these studies, 23 swine (53.3 kg average mass) were impacted at 15 and 30 m/s with a 4.9 kg striker mass with a 150 mm diameter striker plate [30]. According to logistic analysis, VCmax and VmaxCmax were good predictors of the probability of heart rupture and thoracic MAIS > 3, while Cmax was not. Lau and Viano concluded that the viscous criterion is the best indicator for soft tissue injury to many body regions for velocities of deformation of 3–30 m/s [81]. The velocity of impact of automobile occupants to various parts of the automobile interior is in this 3–30 m/s range, which is intermediate to the high velocity pressure waves of a pure blast (in which injury occurs with little compression) and the pure crushing injuries of quasi-static loading (in which injury is due to compression alone). In an analysis of the 39 unembalmed PMHS sternal impacts (average age 62 years) performed by Kroell and others, VCmax of 1.3 m/s was the value for 50 % probability of thoracic AIS > 3, and VCmax of 1.0 m/s for a 25 % probability of AIS > 3, based on Probit analysis [81, 92].
13.6.2.6 Combined Compression and Acceleration Criterion
In 1998 the NHTSA proposed Combined Thoracic Index (CTI) as an injury criterion for frontal impact that combined chest compression and acceleration responses, with the aim of addressing both air bag and belt loading [94].
Where, Amax = Maximum observed acceleration

Aint = Maximum allowable intercept value of acceleration
Dmax = Maximum observed deflection
Dint = Maximum allowable intercept value of deflection
The principles of CTI are explained by the differences in loading of the thorax by belt versus bag systems. For a given load, a belt system would apply greater pressure along its contact area than an air bag system, which has a larger contact area. The chest is more vulnerable to injury under the more concentrated belt loading. With the combined belt/bag system, the predominant loading could range from stiff belt/soft bag which would produce predominantly a line load, to soft belt/stiff bag which would produce a more distributed load. CTI was proposed as a criterion that reflects both the conditions and the various combinations in between. Peak chest acceleration is a measure of the magnitude of total forces applied to the torso, in proportion to the mass of the torso. Chest deflection is an indication of the belt loading contributing to the restraint system effect. The greater the deflection per unit of acceleration, the more the relative contribution of the belt system [94]. Figure 13.7a, b show the cross plot of chest deflection to chest accelerations for different AIS levels and the proposed regulation limits for CTI.
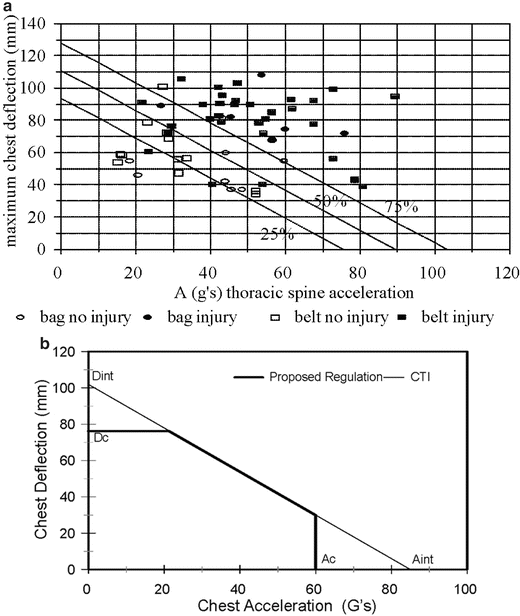
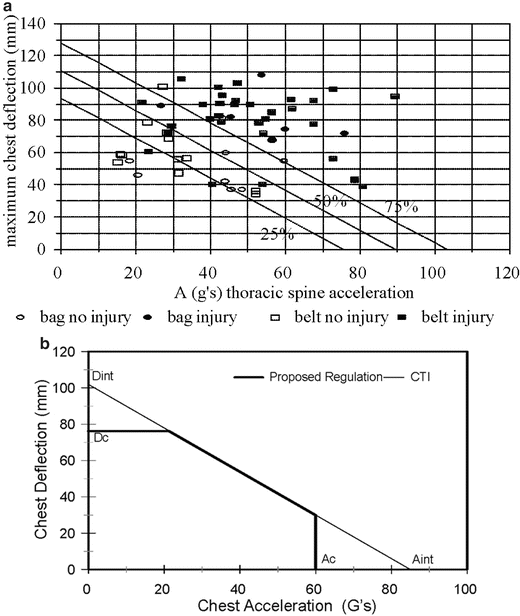
Fig. 13.7
(a) Lines of equal probability of AIS 3 or greater chest injury using the linear combination of maximum deflection and spinal acceleration. (b) Injury criteria for mid-sized adult male Hybrid III dummy (From [94])
CTI was developed based on 71 PMHS tests reported by Kuppa et al. [95]. Five different restraint systems were used: 3-point belt, 2-point belt/knee bolster, 3-point belt/air bag, air bag/knee bolster and air bag/lap belt. Impact velocities ranged from 23 to 57 km/h. Peak g’s in the deceleration profiles ranged from 15 to 25 g’s. Instrumentation included tri-axial accelerometers at T1 and chestbands wrapped around the chest at the location of the 4th and 8th ribs. The 3 ms clip T1 resultant acceleration, maximum chest deflection (Dmax), Vmax and VCmax were analyzed statistically as injury functions using logistic regression analysis of the probability of thoracic AIS of 3 or greater. Univariate and multivariate analyses of the linear combination of these responses were performed. The combination with the best predictive value was -6.43 + 0.076As + 13.68Dmax. The probability of AIS 3 or greater injury using this function is shown in Fig. 13.8. CTI was not incorporated into FMVSS 208 after review and comment to the Notice of Proposed Rulemaking (NPRM). However, stricter criteria for chest deflection were incorporated into FMVSS 208 commencing in model year 2003 as described above under Compression Criteria [88].
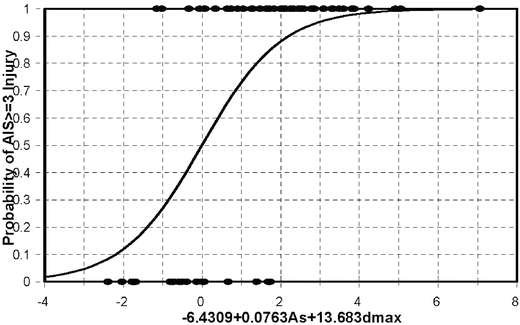
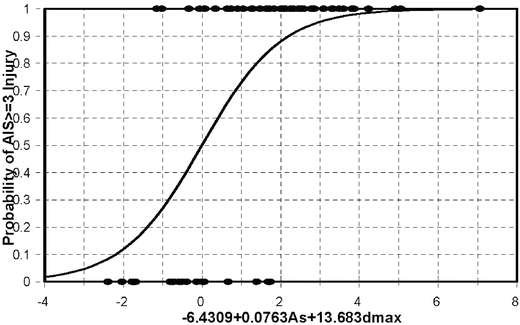
Fig. 13.8
The probability of chest injury in frontal impact using the linear combination of dmax and A as a risk factor (From [94])
13.6.3 Biomechanics of Belt Loading and Combined Restraint Loading
13.6.3.1 Three-Point and Force-Limiting Belt Loading
In a Canadian study of injury to 121 belted occupants, Dalmotas found that shoulder/chest injuries constituted 23 % of AIS 3 and greater injuries [96]. For those drivers who did not contact the steering assembly, injuries were skeletal fractures of clavicle, sternum and ribs in the belt line. No intra-thoracic or abdominal injuries were attributed to belt loading. Patrick and Anderson reported injury results in crash investigations of drivers wearing three-point belts in Volvos [97]. Fourteen of 169 (8.3 %) occupants had rib fractures. Barrier equivalent velocity (BEV) ranged from 1 to 24 m/s. At 0 to 4 m/s, 29 of 32 occupants had no injury. There were 14 rib cage injuries in the 1–24 m/s BEV. Schmidt et al. reported thoracic injury data in a test series with 49 fresh PMHS [98]. Tests were conducted at a crash velocity of 50 km/h and a stopping distance of about 40 cm. Three-point retractor belts were used on 30 and two-point belts plus knee-bar were used on 19 PMHS. Age range was 12–82 years. The fracture patterns followed the belt line and there was an approximate linear relationship between the number of rib fractures and age, with more than 20 fractures occurring in several cases. Injuries to internal organs of chest and abdomen were also documented.
Horsch et al. studied the APR and Volvo real world crash studies relating shoulder belt loading to chest injuries [99]. The analysis of the data indicated that belt loading of the Hybrid III dummy chest resulting in 40 mm chest compression represented about a 25 % risk of AIS 3 or greater thoracic injury. Injury due to belt loading appeared to be driven by chest compression rather than VCmax. In APR and Volvo test data rib fractures increased with age but more so in the APR data base. Crashes severe enough to result in more than 40 mm Hybrid III dummy chest compression were infrequent. Cesari and Bouquet used a diagonal shoulder belt to dynamically load the chest of unembalmed PMHS and dummies [100]. Using two chestbands and an array of linear displacement transducers, the authors determined that the dummy thorax is almost twice as stiff as that of the cadaver. The human thorax had complex deformations with maximum occurring away from the sternum at the lower region of the rib cage.
In 1995 the first generation programmed restraint system (PRS) was introduced in France with seat belt force threshold of 6 kN. Thirty-seven frontal accident cases involving this restraint system were investigated by Bendjellal et al. [101] Analysis of this data showed only two cases were related to AIS 3 level injury but the authors concluded that reducing the shoulder belt force to 4 kN was a necessary further step and required redesign of the air bag. The new system was called PRS II. The PRS II uses a pyrotechnic buckle pretensioner with a 4 kN belt load limiter and air bag deployment to the sides and from the top to bottom in order to reduce risk in out-of-position situations. Foret-Bruno et al. sought to establish a thoracic injury risk function for belted occupants as a function of age and loads applied to the shoulder level [102]. Fifty-percent probability of AIS 3+ occurred at a belt load of 6.9 kN. The authors concluded that a belt load limit of 4 kN combined with a specially designed air bag could protect 95 % of those involved in frontal impacts from AIS 3+ chest injuries. Foret-Bruno et al. compared thoracic injury risks for two occupant populations: belted occupants involved in accidents in which the vehicle was not equipped with a load limiter (378 cases with pyrotechnic pretensioners), and belted occupants involved in accidents in which the vehicles were equipped with 4 or 6 kN load limiters and pyrotechnic pretensioners (347 cases) [103]. A 4 kN load limit resulted in a reduction of thoracic injury risk for all AIS levels, compared to other belt configurations. There was 50–60 % reduction for AIS 2+, 75–85 % for AIS 3+ and a complete absence of AIS 4+ with a 4 kN load limiter.
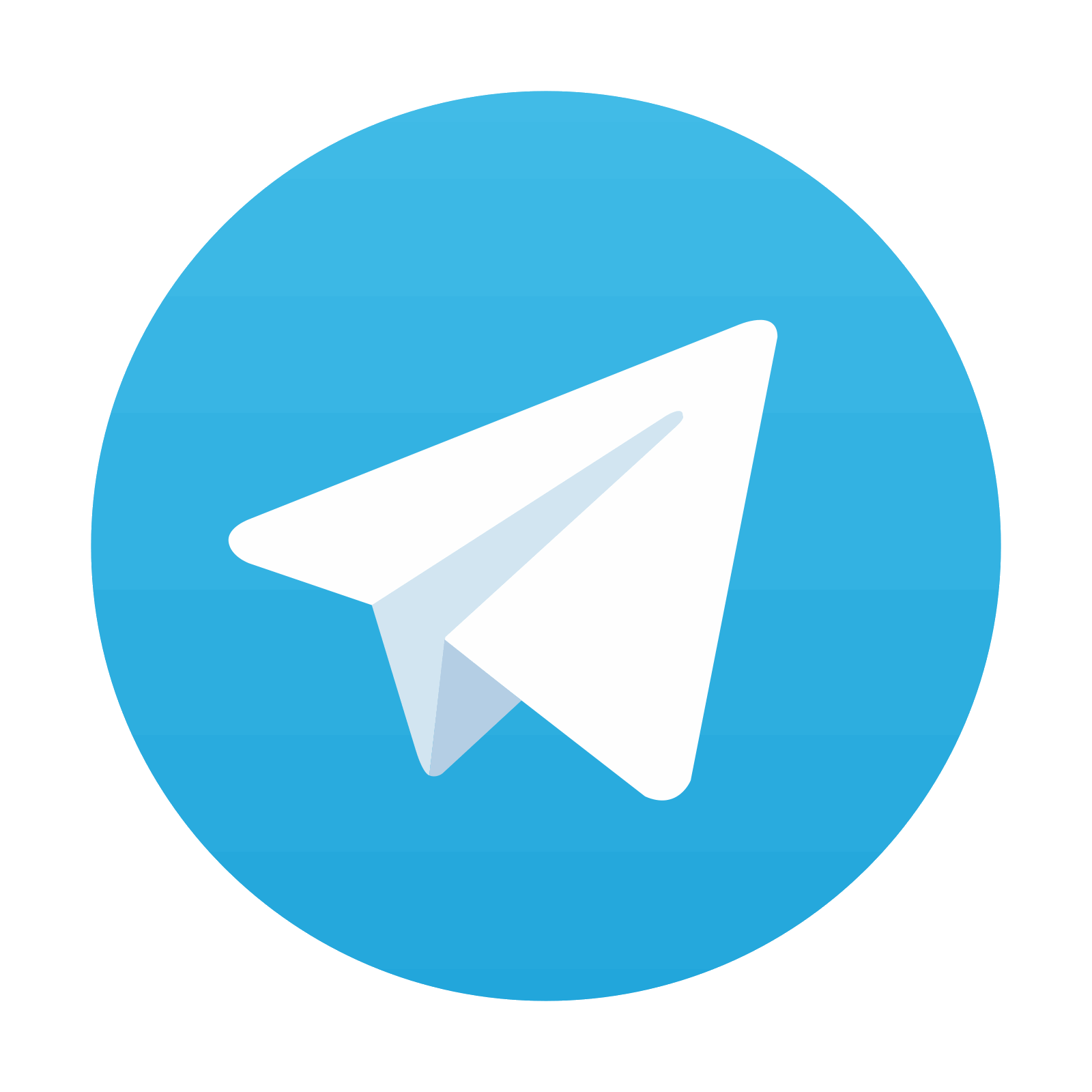
Stay updated, free articles. Join our Telegram channel
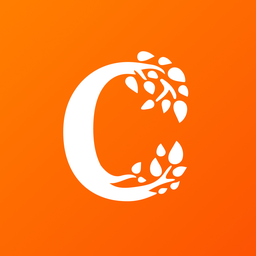
Full access? Get Clinical Tree
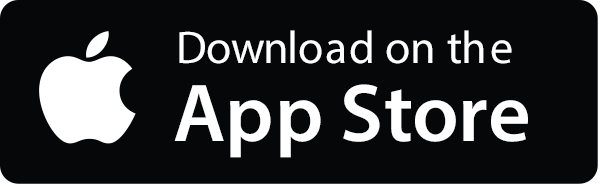
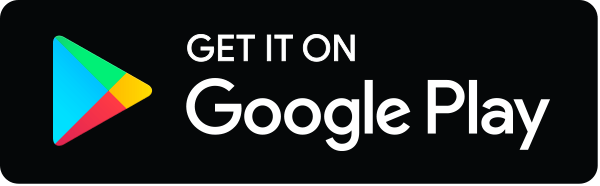