Fig. 20.1
The figure illustrates potential mediators of peripheral sensitization after inflammation. Tissue injury and inflammation lead to the release of various chemicals from non-neuronal cells, including mast cells, macrophages, platelets, immune cells, endothelial cells, Schwann cells, keratinocytes and fibroblasts. These chemicals include protons (H+), purines (adenosine, adenosine triphosphate), nerve growth factor (NGF), cytokines such as tumor necrosis factor (TNF-α) and interleukins (IL-1β, IL-6), leukemia inhibitory factor (LIF), prostaglandin E2 (PGE2), bradykinin, histamine, serotonin (5-HT), platelet activating factor (PAF), and endothelin. Neurogenic mediators, such as SP and CGRP, are released from peripheral nociceptor terminals. The released chemicals may act directly to alter the sensitivity of peripheral nociceptors or indirectly via coupling to one or more peripheral membrane-bound receptors, including transient receptor potential (TRP) channels, acid-sensitive ion channels (ASICs), purinergic (P2X) receptors, G protein–coupled receptors (GPCRs), two-pore potassium channels (K2P), and receptor tyrosine kinase (RTK). Binding of the ligands to these receptors can initiate a cascade of events that includes activation of second-messenger systems (protein kinase A [PKA] and C [PKC]) and alteration of gene regulation (Reproduced with permission from Ringkamp et al. [5])
Central Sensitization: Central sensitization occurs in spinal cord dorsal horn neurons as a result of sustained activation of nociceptors. Neurotransmitters in the dorsal horn include glutamate, N-methyl-D-asparate (NMDA) and SP (a neuropeptide). Neuropeptides can diffuse considerable distances from their site of release because there is no specific reuptake mechanism. Thus, many postsynaptic terminals can be influenced. Peptide levels are significantly increased in persistent pain. Peptides, including substance P, contribute to the excitability of dorsal horn neurons and to the poor localization of some painful conditions [4]. Central sensitization cane be both short term and long term [6] (Fig. 20.2).
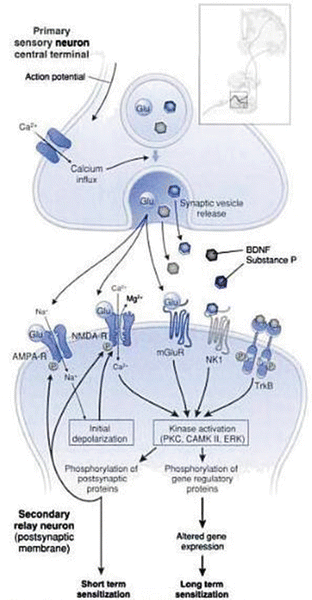
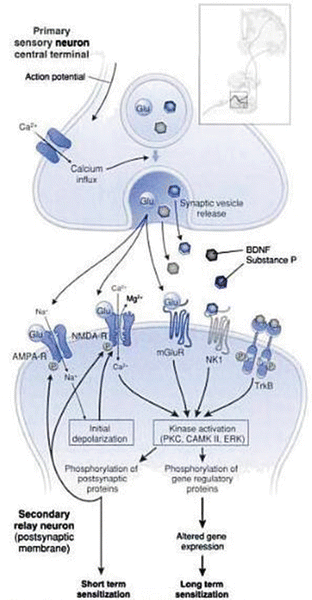
Fig. 20.2
Flow chart displaying the possible outcomes of central sensitization. An action potential from a primary sensory neuron central terminal can lead to both short term and long term sensitization. AMPA-R alpha amino-3hydroxy-5-methyl-4isoxazolepropionic acid receptor; BDNF brain-derived neurotrophic factor; CAMK II calmodulin dependent preotein kinase II; ERK extracellular signal-regulated kinase; NMDA-R N-methyl-D-aspartate receptor; PKC protein kinase C (Reproduced with permission from Golan et al. [6])
Pain generators in the spine include the intervertebral discs, paraspinal muscles, facet joint capsules, dorsal root ganglia, nerve roots and dorsal horn [7]. Disc, muscle and facet joint capsules all contain nociceptors that can be a source of peripheral sensitization if these tissues are injured or inflamed. The dorsal roots and dorsal root ganglion are sources of neuropathic pain which is described later in this chapter [7] (Fig. 20.3).
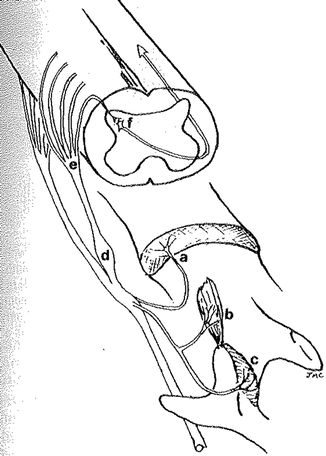
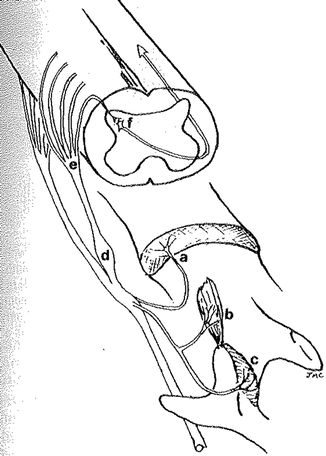
Fig. 20.3
Diagram showing various pain generators in the spine. These include: intervertebral discs (a); paraspinal muscles (b); facet joint capsules (c); dorsal root ganglia (d); nerve roots (e); dorsal horn (f) (Reproduced with permission from Cavanaugh [7])
20.2 Biomechanics of Noxious Loading of Thoraco-lumbar Spine
20.2.1 Injury Tolerance of the Thoraco-lumbar Spine
+Gz acceleration (upward vertical acceleration to the torso and spine) occurs in ejection from aircraft seats, falling from a height onto the buttocks or vehicle occupant loading of the seat pan during vertical or frontal impact of the vehicle. Vertical loading occurs when explosive devices deploy under a vehicle and cause the vehicle to accelerate skyward. Such is the case when improvised explosive devices (IEDs) were deployed against coalition forces in the recent wars in Iraq and Afghanistan.
20.2.1.1 Volunteer Data from Servicemen
Eiband reported that with lap, shoulder straps and face curtain in use, 16 g’s for 0.04 s has been endured in catapult seat experiments. A 20 g median value has been assumed as a safe limit for ejection seat performance since World War II at pulse duration of 0.005–0.5 s. Hogs subjected to 110 g’s for 0.002 s recovered within a few days. Onset rate to g level (g’s per second) is termed jolt. 500 to 1300 g’s per second has jolted the subject severely but without permanent injury. A jolt of 115–180 g’s per second is considered preferable [8].
The type of torso support is critical in regards to thoraco-lumbar spine injury tolerance. The minimum support required to avoid this was lap and shoulder belts in the review by Eiband [8]. Use of the armrest increases vertical loading tolerance further by reducing the load on lumbar vertebrae. Providing support for the head and neck reduces the severe neck flexion that can occur in sudden vertical loading [8].
20.2.1.2 Cadaver Studies
Ewing et al. tested embalmed cadavers in hyperextended, erect and flexed modes in +Gz acceleration. In the hyperextended mode g-level to fracture averaged 17.6 g, compared to 10.4 g in erect and 9.0 g in flexed mode [9].
Vulcan et al. reported that the CG of the body lies 0.75–1.45 in. anterior to the centerline of the T9 vertebra. In four cadavers vertebral bodies were strain gauged at T11 to L4. In cases where the shoulder was allowed to rotate forward there was a peak in the vertebral strain levels that corresponded to peak shoulder strap loading. If the shoulders were held back with tight straps from flexing forward the large vertebral strains were avoided. Thus, upper body flexion plays a key role in stresses of the lower vertebrae under sudden vertical loading [10].
Prasad et al. showed that the facets share part of the loading during hyperextension. In the erect mode the facet capsular ligaments go into tension, causing the vertebral bodies to sustain more compressive load than the total spine load [11]. In the hyperextended mode the facets relieve the vertebral bodies of some of the compressive load. Yang and King showed that the mechanism of load transmission is by the bottoming of the tip of the inferior facet onto the lamina below [12].
In summary, thoraco-lumbar spine injury tolerance increases as the spine posture changes from flexion to hyperextension. As the body becomes more flexed and trunk support is removed, this tolerance level is reduced. Vertebral wedge fractures can occur when there is a flexion component in addition to the Gz loading. In severe cases of compressive loading, burst fractures with retropulsion of vertebral body fragments into the spinal canal can occur, causing spinal cord and/or nerve root injury.
20.2.1.3 Gx Acceleration (Sternum to Spine Acceleration as Occurs in Frontal Impacts)
Using a 2D math model Prasad and King reported that the lumbar vertebrae could undergo compression in a frontal impact. It was hypothesized that the spine attempted to straighten out during -Gx acceleration, loading the lumbar spine [13]. This was supported in a study by Begeman et al who measured large seat pan loads in cadaver tests in which the upper torso was restrained [14].
Miniaci and McLaren attributed four anterolateral wedge fractures to the wearing of lap-shoulder belts in motor vehicle accidents [15].
20.3 Joint, Ligament and Skeletal Pain
A diagram of the anatomy of thoraco-lumbar motion segments is illustrated in Fig. 20.4 [16]. Mechanisms by which individual structures of the lumbar spine can be sources of pain are described below.
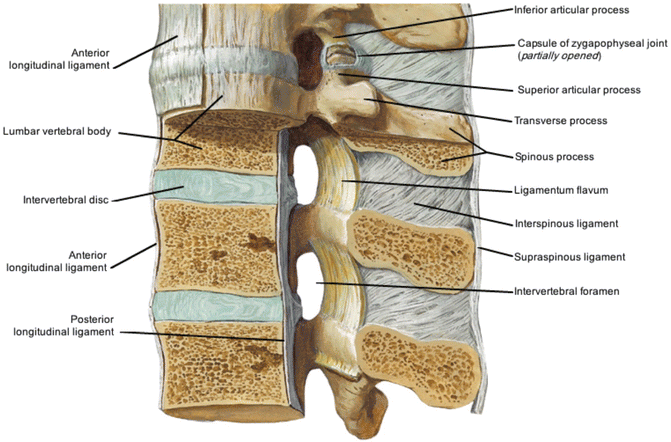
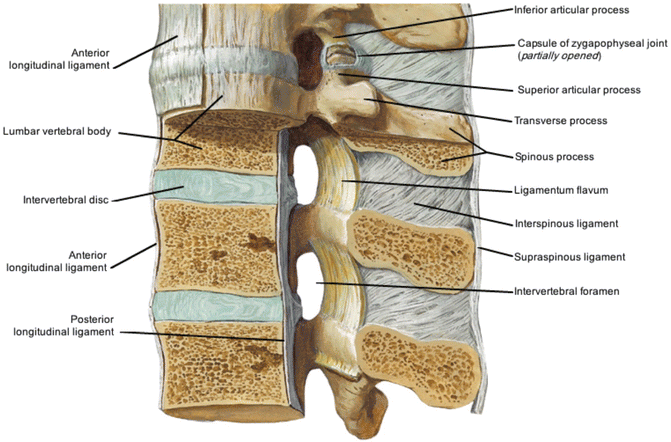
Fig. 20.4
The motion segments of the lumbar spine and accompanying ligaments (Reproduced with permission from Freemont [16])
20.3.1 Intervertebral Discs
20.3.1.1 Disc Herniation
The herniated (ruptured) intervertebral disc can be a source of pain. The disc outer annulus contains nerve endings that can transmit signals that lead to pain. Degenerated discs have pain fibers that extend further into the disc than in healthy discs. In addition, the nucleus pulposus, the gel-like material that can extrude out of a ruptured disc can cause inflammation that sensitizes nerve endings to cause low back pain and nerve roots to cause sciatica.
The disc is stronger in vertical loading than are the vertebral bodies. The vertebral bodies will usually fail first. Disc ruptures from a single loading event are highly unlikely based on biomechanical studies. Henzel et al. observed that early researchers noted that the vertebral body broke before the adjacent disc incurred any damage [17]. Yoganandan et al. tested individual discs and vertebral bodies and noted that thoracic and lumbar vertebral bodies had failure loads of 2,642–4,590 N, healthy discs 11,030 N and degenerated discs 5,300 N on average [18]. Brinckmann performed a study in which 25 PMHS lumbar intervertebral discs had the annular fibers from the inside out, preserving ½ to 1 mm of the outer annulus. The motion segment was then loaded to 1,000 N (225 lb). There were no disc ruptures, bulging was minimal and there was never extrusion of disc material. Disc ruptures did not occur even after the vertebral body was loaded to failure [19].
Adams and Hutton were able to produce disc ruptures in the case of extreme flexion combined with high compressive loads [20]. Sixty-one joints were tested to failure. Twenty-six failed by prolapse (rupture) of the intervertebral disc. The average compressive force to produce rupture was 5,448 N and the average flexion angle 12.8° between two vertebrae. This angle would represent a flexion of over 60° in five vertebrae, an extreme condition beyond the physiologic range. Thus, hyperflexion between lumbar vertebrae and high compressive loading are conditions that have produced disc rupture experimentally. However, other studies indicate the vertebrae will fracture at these loads before the disc ruptures.
Gordon et al. produced disc rupture by loading 14 human cadaveric lumbar motion segments (two vertebrae plus the disc) at 1.5 Hz for an average of 6.9 h, in a combination of 7° of flexion, 3° of rotation and 1,334 N of compression. Ten discs failed through annular protrusions. The average number of cycles to failure was 36,750. This study supports repetitive loading over time as a primary cause of disc herniation [21].
Callaghan ad McGill noted that while intervertebral disc herniations are observed clinically, consistent reproduction of this injury in the laboratory has been elusive [22]. They performed a study designed to examine the biomechanical response and failure mechanics of spine motion segments to highly repetitive low magnitude complex loading. They tested porcine cervical spine motion segments (C3–C4) in applied axial compressive loads with pure flexion/extension moments. Dynamic testing was conducted to a maximum of 86,400 bending cycles at a rate of 1 Hz with simultaneous torques, angular rotation and axial deformations recorded for the duration of the test. The results supported the notion that intervertebral disc herniation may be more linked to repeated flexion extension motions than applied joint compression, at least with younger, non-degenerated specimens.
In summary, herniation of a lumbar disc from a single accident is not impossible but highly unusual. It has been replicated in an extreme combination of compression and flexion, as shown by Adams and Hutton. Disc rupture is typically a wear-and-tear phenomenon that occurs over thousands of loading cycles and is not the result of a single loading event. Loads high enough to rupture a disc will more likely fracture a vertebral body first.
20.3.1.2 Disc Innervation and Pain
Clinically the role of degenerating intervertebral discs and the consequences of ruptured disc in the ensuing symptoms of low back pain have been well documented. A recent review by Bogduk et al. suggests that discogenic pain can occur and be diagnosed under strict operational criteria [23]. For an anatomical structure such as intervertebral disc (IVD) to be a source of pain, a nerve supply is required. One of the earliest citations on IVD innervation in relationship to back pain dates back to a report by Wiberg [24]. However interest in disc innervation predates this as indicated by studies of others in support of IVD innervation [25, 26] with one of the prior studies of the time reporting no nerve elements in humans discs but in the surrounding ligaments [27]. This lack of nerve fibers in disc annular region was again reported [28]. During later years, Mulligan reported disc annulus innervation using a canine thoracic vertebral column [29]. A previous study by Stilwell reported unmyelinated nerve fibres with free nerve endings being confined to the superficial lamina of loose connective tissue of the annulus [30]. However, Yoshizawa showed profuse non-myelinated axonal networks and free nerve terminals in the outer (lateral) half of the annulus of IVDs removed for low back pain [31]. In one of the subsequent anatomical studies Bogduk and colleagues offered irrefutable evidence of nerve supply to human lumbar IVD. They described that branches of sinuvertebral nerve innervate the posterior aspect of the IVDs and that the posterolateral aspects of the discs receive branches from adjacent ventral primary rami and from the grey rami communicantes near their junction with the ventral primary rami [32, 33] (Fig. 20.5A). Subsequently, Kojima et al. described that sinuvertebral innervation to posterolateral portion of the annulus fibrosus (AF) in rat lumbar IVD and indicated no nerve fibers and terminals in deep layer of the AF or the nucleus pulposus [34]. Using a retrograde transport method, Morinaga et al. showed IVD afferent innervation in rat specimens. They demonstrated that the anterior portion of the L5–L6 lumbar IVD was innervated from L1 or L2 spinal nerves and suggested this as a possible explanation for why patients with lower lumbar disc lesions sometimes complain of inguinal pain corresponding to the L1–L2 dermatome [35]. Using a similar approach, Ohtori et al. showed that that the dorsal portion of the L1–L6 discs of rats was shown to be multi-segmentally innervated by the T11 through L6 dorsal root ganglia using paravertebral sympathetic trunks and sinuvertebral nerves as nerve pathways [36, 37]. Nevertheless, the data on disc innervation from rodent studies needs to be interpreted cautiously in cases of human discogenic pain.
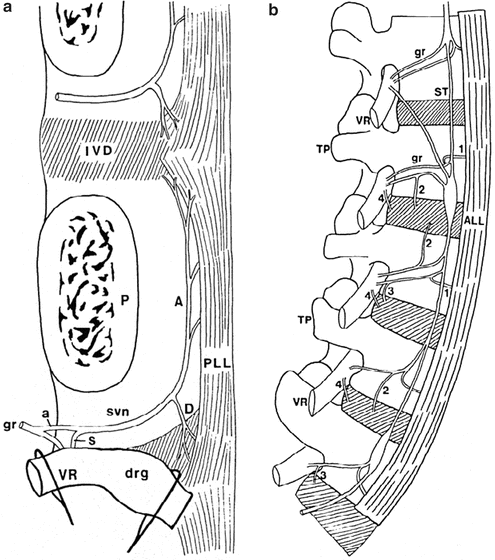
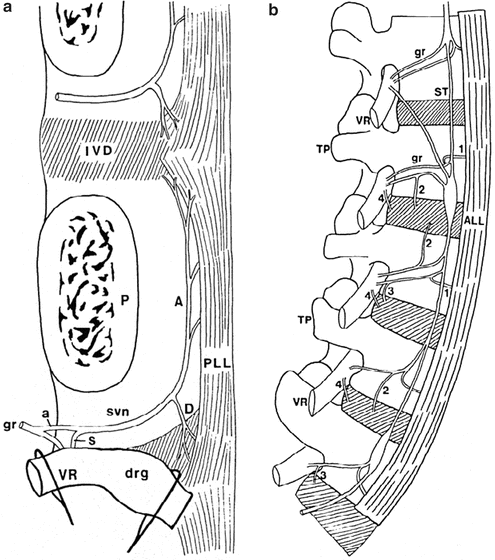
Fig. 20.5
(a) Diagram of a left lumbar sinuvertebral nerve (svn) with pedicle of the vertebra (P) transected and the neural arch and the dural sac removed. The ventral ramus (VR) and dorsal root ganglion (drg) are retracted to reveal the origins of the sinuvertebral nerve from a somatic root (s) from the ventral ramus, and an autonomic root (a) from the grey ramus communicans (gr). Ascending (A) and descending (D) branches innervate the posterior longitudinal ligament (PLL) and intervertebral discs (IVD) at the level of the foraminal entry of the nerve and at the next level above. (b) A diagram of nerves innervating the anterior and lateral aspects of the lumbar vertebral column. TP transverse process, ALL anterior longitudinal ligament, VR ventral ramus, ST sympathetic trunk, gr grey rami communicantes. 1 are branches to anterior longitudinal ligament, 2 are branches to lateral aspects of intervertebral disc, 3 are branches to intervertebral disc from grey rami, 4 are branches to intervertebral disc from ventral rami (Reproduced with permission from Bogduk [33])
Immunohistochemical evidence for IVD innervation was supported using antibodies to protein gene product (PGP 9.5), a general neuronal marker. Nerve fibers containing PGP 9.5 were observed in the outer annulus running between and across the collagenous lamellae in human disc specimens [38]. Palmgren et al. on the other hand showed PGP 9.5 reactive nerve fibers penetrating 3.5 and 1.1 mm into the annulus in normal human IVD specimens, whereas Willenegger et al. showed PGP 9.5 immunoreactive nerve fibers in the periphery of the IVD in sections from dogs [39, 40]. In an earlier study Cavanaugh et al., using a silver impregnation method, showed numerous fine profiles of nerve fibers limited to the superficial anulus in rabbit lumbar IVD [41].
The IVDs have also been reported to have various types of nerve endings. Roofe reported naked nerve endings terminating in annulus and posterior longitudinal ligament [25]. Stilwell reported unmyelinated nerve fibers with free nerve endings [30]. Simple free nerve endings were also reported by Hirsch et al. and Jackson et al. [42–44]. Malinsky found five types of free nerve endings in the outer annulus layer: lone, simple, free; branching; shrubby; mesh-like loops, and clusters running in parallel. Malinsky also found encapsulated and partially encapsulated nerve endings on the external lateral annulus surface [45]. The presence of nerve endings was further confirmed by Roberts et al. who reported mechanoreceptors resembling Pacinian corpuscles, Ruffini endings, and, most frequently, Golgi tendon organs in the outer 2–3 lamellae of the IVD and associated anterior longitudinal ligament (ALL) from human and bovine specimens [46]. Ruffini, Golgi type and free nerve endings were also reported by others in the superficial layers of the annulus and they suggested that the anterior part of the disc has a greater frequency of encapsulated receptors [42].
Mooney reviewed much of the current knowledge on low back pain and concluded that the disc may be primary source in the production of low back pain, but the mechanisms of pain production are uncertain [47]. Hisrch et al. reported that 0.3 ml (11 %) of hypertonic saline injected into the disc produced severe pain, ‘identical to a real lumbago’, that could not be localized but that was described as a deep aching across the low back [43]. Kuslich et al. demonstrated that of 144 patients, whose central lateral annulus was stimulated, 71 % had pain by the procedure and 30 % experienced significant pain. In this study, 18 spine tissue sites were stimulated with blunt surgical instruments or electrical current of low voltage. Significant pain was reported by 90 % of patients upon stimulation of central lateral annulus, and by 15 % upon central annulus stimulation [48]. In a retrospective study of a cohort of 28 consecutive patients, De Palma et al. concluded that outer annular fissures were a source of discogenic pain attributed to sensitized nociceptors in annular tears. The subjects underwent provocation diskography and analgesic diskography utilizing a balloon-tipped intradiskal catheter allowing intradiskal injection of anesthetic. Eighty percent of painful intervertebral disks as detected by provocation diskography were sufficiently anesthetized, resulting in >50 % reduction in low-back pain during analgesic diskography [49].
The disc is made up of an inner gelatinous nucleus pulposus which is confined above and below by the end plates of the vertebral bodies and circumferentially by the fibres of the thick annulus fibrosus (AF) [16] (Fig. 20.4). The cells of the annulus are fibroblast-like and the cells of the nucleus pulposus (NP) are chondrocyte–like [50]. The Chondrocyte-like cells of the NP synthesize type II collagen, proteoglycans, and non-collagenous proteins that form the matrix of the nucleus pulposus and the cartilage endplate. Fibroblast-like cells synthesize type I and type II collagen for the annulus fibrosus [51]. The disc material undergoes constant turnover by getting degraded by matrix metalloproteinases (MMPs) secreted by the chondrocytes [52, 53]. Furthermore, disc degeneration and a persistent state of inflammation may arise from synthesis of abnormal disc components, or an increase in synthesis of mediators such as nitric oxide, interleukins, prostaglandin E2, and matrix metalloproteinases [54–58] that can lead to matrix degradation. It has also been suggested that the number of nerve fibers increases following disc degeneration that innervate not only the outer AF and also the inner nucleus pulposus. Also increased are the number of mechanoreceptors in the superficial layers of the disc [59]. Furthermore, a recent investigation reported that increased transcription growth factor beta (TGF-β) and SP levels were predominantly found in chronic degenerative disc disease (DDD) with MMP3 increased in acute herniated disc material suggesting variations in the expression of mediators between acute and chronic processes [60]. Another investigation by Richardson et al. showed that nerve growth factor (NGF) significantly correlated with both MMP-10 and substance P mRNA in degenerate NP samples and suggested that MMP-10 expression increases in the symptomatic degenerated IVD [61].
Experimentally, the potential role for disc in pain generation comes from some recent studies that showed animals with epidural presence of nucleus pulposus or disc puncture showing behavior of pain [62, 63]. Behavioral and histological signs of alleviation by pharmacological intervention by using tumor necrosis factor (TNF) inhibitor [64–66] were also reported. Neurophysiologically, the released disc material is capable of inducing functional changes in adjacent exposed nerve roots as evidenced by reduced conduction velocity [67, 68]. It has been suggested that the leakage of disc material from annular tears to adjacent nerve roots is one pathophysiologic mechanism of low back pain following disc degeneration. The role of other factors such as ageing, genes, nutrition, toxic factors, metabolic disorders, and various mechanical factors has also been reviewed by others [51, 16]. It is suggested that pressure and chemical irritation of nociceptive nerves is dependent on degenerated discs or their material exciting sensory neural elements, especially in the posterior longitudinal ligament and possibly also in the peripheral parts of the annulus fibrosus [69]. It was also suggested that interleukin 1 beta (IL-1β) is generated during IVD degeneration, which stimulates the expression of vascular endothelial growth factor (VEGF), nerve growth factor (NGF), and brain derived growth factor (BDNF), resulting in angiogenesis and innervation [70]. It is also plausible that the released trophic factors can act on nociceptive nerve fibers and also contribute to further release of inflammatory mediators [59, 71] (Fig. 20.6).
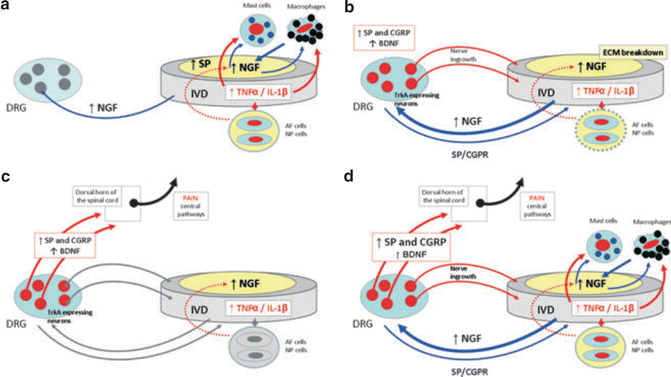
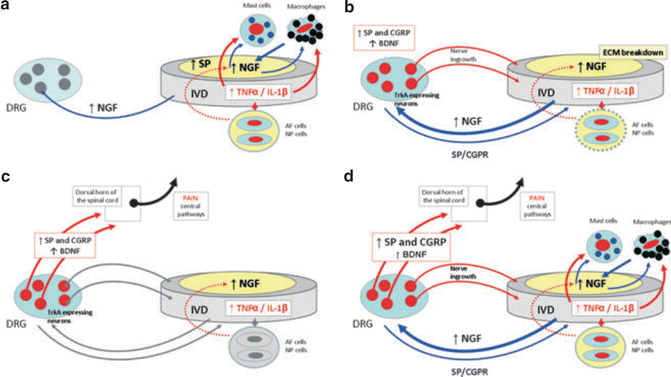
Fig. 20.6
Schematic representation of the potential mechanisms involved in the genesis of the discogenic pain. (a) Inflammation causes release of proinflammatory cytokines in the intervertebral disc (IVD), which act on mast cells and macrophages to trigger secretion of NGF. Cells in the IVD upregulate expression of NGF and substance P (SP). Increased levels of NGF can be retrogradely transported to dorsal root ganglia (DRGs) or stimulate mastocytes and macrophages locally initiating a positive feedback loop. (b) Increased levels of NGF reaching the DRGs act on TrkA-expressing neurons inducing expression of peptides that mediate pain [SP and calcitonin gene-related peptide (CGRP)]. The increased levels of NGF in the IVD, as well as the breakdown of the IVD aggrecans, result in ingrowth of nociceptive nerve fibers. Anterograde transport of SP and CGRP to the IVD also may occur to maintain pain. (c) Synaptic transmission in lamina I and II of the dorsal horn of the spinal cord is mediated by SP and CGRP. In addition, brain-derived neurotrophic factor (BDNF) produced in DRG is released to these laminas and modulates pain transmission. (d) It is proposed that these complex networks are able to originate and maintain pain of IVD origin (Reproduced with permission from Garcia-Cosamalon et al. [59])
20.3.2 Anterior Longitudinal Ligament (ALL)
The anterior longitudinal ligament (ALL) runs on the anterior (ventral) surface of the spine traversing the vertebral bodies and intervertebral discs (Fig. 20.4) [16]. Similar to the IVD, nerve supply to ALL is controversial with very limited studies supporting a less developed innervation of ALL. Bogduk performed one of the earliest investigation of ALL innervation and reported that they were innervated by the branches of the grey rami communicantes [33, 32] (Fig. 20.5B). In subsequent work, Sato et al. performed a detailed investigation of the nerve supply to ALL. They reported that the ALL receives nerve branches from the sympathetic trunk and splanchnic nerves non-segmentally and suggested that the sympathetic nerves may be involved in proprioception of the spinal column [72]. The presence of nerve fibers in ALL was further supported by immunohistochemical studies of Kallakuri et al. who reported dense network of PGP 9.5 reactive nerve fibers. They also reported SP and calcitonin gene related peptide (CGRP) reactive nerve fibers in ALL supporting a putative nociceptive role for this tissue [73]. The presence of mechanoreceptors in ALL was reported by Roberts et al. and suggested that they may play a role in sensing posture, movement and nociception [46]. Clinically by a rare case of heterotopic ossification (HO) in the plane of ALL, symptoms of low back pain due to a fracture in HO was also reported [74] suggesting a role for ALL in mediating LBP.
20.3.3 Posterior Longitudinal Ligament
The posterior longitudinal ligament (PLL) is located within the vertebral canal and extends along the posterior aspect of the vertebral bodies from the body of axis to the sacrum. It is narrow at the level of vertebral body and is wider at intervertebral discs (IVDs) [16] (Fig. 20.4). The nerve supply to the longitudinal ligaments has been reported previously by several investigators [25, 29, 44, 75–76]. Bogduk in one of the earliest neuroanatomic investigations revealed that the PLL is also innervated by branches of sinuvertebral nerves and grey rami communicantes [32, 33] (Fig. 20.5A). These findings were further supported by Higuchi and Sato who also reported PLL innervation from sinuvertebral nerve via the branches of the deep transverse rami of the rami communicantes [72]. Kojima using the acetylcholinesterase (AchE) enzyme histochemistry offered further details on PLL innervation in rat. They reported that the meningeal branch of the spinal nerve (the sinuvertebral nerve) enters the vertebral canal and divides into ascending and descending branches which fuse with those from adjacent vertebrae. They give off transverse branches, connecting with those from the opposite side to form the superficial nerve fiber network in the intervertebral segment, which spreads to the vertebral segment of the PLL. Apart from this superficial nervous network, many nerve fibres enter through the posterolateral portion of the annulus fibrosus (AF) and form a dense, fine nerve fiber network in the deep layer of the intervertebral portion of the PLL and the superficial layer of the disc annulus [34]. Cavanaugh et al. using a silver impregnation technique further supported PLL innervation in close association with AF of IVD in rabbits with occasional encapsulated endings in the annular surface and PLL [41].
One of the earliest evidence of PLL neuropeptide innervation comes from a study by Korkala et al. who showed SP reactive nerve fibers in human PLL samples collected during surgery [78]. Later, Konttinen et al. showed SP and CGRP reactive fibers that often co-localized with cytoskeletal neurofilaments in the PLL of human lumbar disc specimens [69]. Ahmed et al. reported peptidergic (neuropeptide Y, NPY and vasoactive intestinal polypeptide, VIP) and noradrenergic (tyrosine hydroxylase, TH) immunoreactive nerve fibers in the spinal ligaments in preparations of rat lumbar spine [79]. Imai et al. using CGRP and TH as markers reported that the lumbar PLL was dually innervated with one system as polysegmentally innervated and closely associated with autonomic innervation and the other one as unisegmentally innervated and not associated with autonomic fibers [80]. von During and colleagues showed PGP 9.5 reactive nerve fibers with the PLL in the region of IVD being rich in capillaries forming a dense plexus within the ventral part and extending to the outer AF [81]. Kallakuri et al. show further evidence of PGP 9.5 reactive network of large and medium sized bundles and small diameter fibers. They also reported PLL nerve fibers reactive to SP, CGRP and some showing nicotinamide adenine dinculeotide phosphate (NADPH)-diaphorase activity [73]. Yahia and colleagues in their scanning electron microscopy (SEM) study reported PLL innervation both in the superficial and deep layers using neurofilament protein (NFP) immunohistochemistry and suggested that most of the nerve fibers terminated as simple free endings that can act as nociceptors [82]. In neurophysiology studies in the cat, mechanosensitive afferent units in the lumbar posterior longitudinal ligament were reported. The majority of the units were located around the intervertebral disc level with conduction velocities of the units in the range of Group III (0.5–2.5 m/s) and Group IV (2.5–20 m/s) with putative nociceptive function [83].
20.3.4 Dural Innervation
The current literature on dural innervation is very limited, with variations on the extent of innervation of dorsal and ventral aspects [79, 84–87]. The dorsal aspect of the dura is considered to be less densely innervated than the ventral aspect. Groen et al. offered some detailed observations on dorsal dural innervation in studies conducted on human fetal tissue using acetylcholinesterase staining. They described the nerves on the dorsal dura as small in number without forming a plexus and not reaching the medial region of the dura [86]. However, immunohistochemical studies in rabbits by Kallakuri et al. have shown that dorsal dura has small bundles of PGP 9.5 reactive nerve fibers forming connections with adjacent nerve fibers. Many nerves ran longitudinally and perpendicularly and extended large distances on the surface, with nerve fibers reaching midline occasionally in rabbit lumbar dural preparations. The authors further showed prominent SP, CGRP and TH immunoreactive as well as NADPH diaphorase reactive nerve fibers on the dorsal aspect of the dura. The CGRP, TH, and NADPH diaphorase reactive fibers were described as being restricted to dural sleeve on the lateral margins [73]. Furthermore, Ahmed et al. have also reported the presence of NPY-, TH- and VIP-positive fibers in the dorsal aspect [79]. SP and CGRP immunoreactive fibers on the lumbar dorsal dura was further confirmed by a more recent investigation which also suggested that the density of SP and CGRP nerve fibers increases following laminectomy [88]. Thus, pain originating in the dura may be related to the sensitization of various nociceptive nerve fibers. Intradural disc herniation and dural erosion are themselves rare [89].
Innervation of the ventral aspect of the dura has been more extensively reported [30, 77, 86, 87, 90–92]. Groen et al. through their work on human fetuses using acetylcholinesterase staining described that ventral dura receives innervation from the sinuvertebral nerves, the nerve plexus of the posterior longitudinal ligament as well as the plexus of the radicular branches of segmental arteries. They also reported the nerves may extend up to eight segments with overlap between adjacent nerves and attributed this as possible explanation for extrasegmentally referred dural pain [86]. Kallakuri et al. reported PGP 9.5 reactive nerve fibers extending throughout the rabbit ventral dura matrix and suggested a multisegmental innervation on the ventral aspect. Similarly, they also reported prominent SP, CGRP, TH reactive and NADPH diaphorase reactive nerve fibers on the ventral dura [73] (Fig. 20.7). Neuropeptide (NPY, TH and VIP) reactive nerve fibers in the dura were also reported by others [79, 84]. Kumar et al. concluded the SP and CGRP innervation of the lumbar spinal dura was not dense [85]. Furthermore, an extensive network of acetylcholinesterase containing nerve fibers has been reported in rat spinal dura [93].
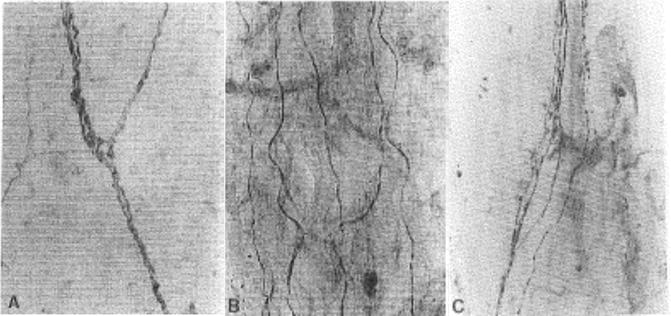
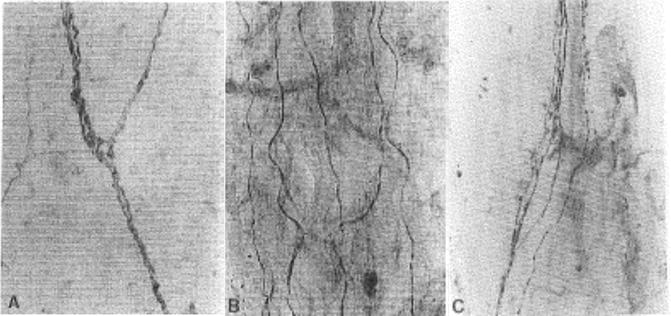
Fig. 20.7
Innervation of dura and the longitudinal ligaments. Immunoreactive fibers to protein gene product 9.5 (PGP 9.5), a general neuronal marker were demonstrated in dorsal dura (a) and posterior longitudinal ligament (b). PGP 9.5 immunoreactive fibers were also shown in ventral dura and anterior longitudinal ligament. Figure (c) shows SP reactive nerve fibers in PLL. Such nerve fibers were also shown in the dura and ALL (Reproduced with permission from Kallakuri et al. [73])
20.3.5 Ligamentum Flavum
There is very little description on the nerve supply to the ligamentum flavum. Ligmentum flava connect the lamina of adjacent vertebra. On a macroscopic level, this tissue has superficial and deep layers whose fibers are opposite and form close connections with the tendons of some spinal erector muscles. Microscopically, the ligamentum flavum reveals prominent elastic fibers [94]. Although studies by Vandenabeele et al. [95] and Ashton et al. [96], offer no support for the presence of nerve fibers, Bucknill and colleagues in a recent immunohistochemical investigation offered definitive evidence of nerve fibres in ligamentum flavum as shown by PGP 9.5 immunoreactivity in samples harvested from low back pain patients. They further showed the presence of sodium channel (SNS/PN3 and NaN/SNS2) immunoreactivity in a subset of nerve fibers of the ligamentum flavum [97]. The presence of ligamentum flavum innervation is consistent with the previous observations of Rhalmi and colleagues who showed neurofilament immunoreactive nerve fibers close to blood vessels and fat globules [98]. It has been suggested that the bulging of the ligamentum flavum contributes to narrowing of the spinal canal which may also have potential implications in the genesis of painful spine conditions [99]. Furthermore, it has also been suggested that a hypertrophic ligamentum flavum may contribute to nerve root compression at the level of the lateral spinal recess and these hypertrophied parts needs to be removed completely during surgical decompression [100]. Also, ligamentum flavum hematoma can compress the adjacent nerve root and in turn contribute to painful conditions [101–103].
Summary: The above review offers extensive evidence in support of nociceptive neuropeptide innervation of the longitudinal ligaments (anterior and posterior), the spinal dura mater and the ligamentum flavum. Considering the important and well-established roles these neuropeptides (SP and CGRP) play in inflammation and pain, it plausible that these structures can be a source of pain. This may particularly be crucial in the case of PLL and ventral dura, considering their close proximity to the IVD. Release of inflammatory mediators from degenerated discs [67, 68] can potentially affect the neural elements in these tissues, leading to altered sensation and pain.
20.3.6 Facet Joints
The morphology of the lumbar facet capsules was studied by Yamashita et al. Via microscopic examination of cadaveric specimens the facet capsules were found to have an outer tough ligamentous layer and a softer inner elastic layer [104].
Nerve supply to lumbar facets occurs via the medial branches of the dorsal rami [33] (Fig. 20.8). The L1–L4 dorsal rami divide into medial and lateral branches near the transverse process. The medial branch travels medially around the base of the superior articular process and under the mamillo-accessory ligament. The medial branch divides into articular branches, supplying the facet joints above and below. Medial branches also supply multifidus and interspinous muscles and the interspinous ligament. The L5 medial articular branch supplies the L5–S1 facet joint and multifidus muscle [33].
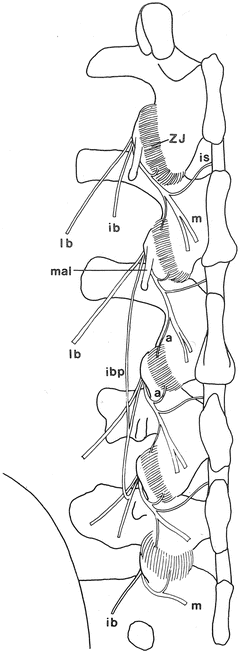
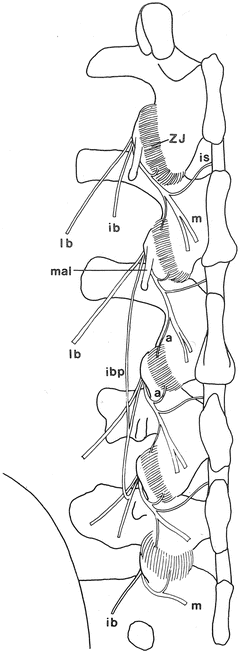
Fig. 20.8
A diagram of the dorsal view of the branches of the left lumbar dorsal rami. Mamillo-accessory ligaments (mal) have been lifted in-situ covering the L1 and L2 medial branches. ZJ zygapophysial joint, m medial branch, lb lateral branch, ib intermediate branch of the dorsal rami, ibp intermediate branch plexus, is interspinous branch, a articular branches of the dorsal rami (Reproduced with permission from Bogduk [33])
To treat facet pain, facet injections as well as medial branch denervation have been carried out and reported by several investigators. Schwarzer et al. injected lumbar facet joints of 146 patients with non-specific low back pain and no definitive radiologic findings. Fifteen percent of these patients had pain relief with a short acting anesthetic (lidocaine) and longer pain relief with a longer acting anesthetic (bupivacaine) [105].
Kuslich et al. reported on 193 awake patients undergoing surgery for disc herniation and/or spinal stenosis. On these patients progressive local anesthesia was performed with 1 % lidocaine. Fifty-four percent of patients had some sensation when the facet capsule was stimulated and 20 % had significant pain [48].
Manchikanti et al. carried out a retrospective analysis of diagnostic facet joint injections in 424 spinal pain patients, divided into 6 age groups. The prevalence of cervical facet joint-related pain was 33–42 % and similar between age groups. The prevalence of lumbar facet joint pain ranged from 18% to 44 % and was higher in older age groups [106].
Eubanks et al. examined a total of 647 cadaveric lumbar spines for evidence of lumbar facet arthrosis. Grading ranged from 0 to 4 (from no arthritis to complete ankylosis). Facet arthrosis was present at the following vertebral levels: 53 % (L1–L2), 66 % (L2–L3), 72 % (L3–L4), 79 % (L4–L5), and 59 % (L5–S1). By decade, facet arthrosis was present in 57 % of 20- to 29-year-olds, 82 % of 30- to 39-year-olds, 93 % of 40- to 49-year-olds, 97 % in 50- to 59-year-olds, and 100 % in those >60 years old [107].
Beaman et al. examined 14 facet joints from patients with low back pain. All facets exhibited cartilage surface irregularity and fibrillation. Erosion channels had progressed into subchondral bone and substance P positive fibers were observed within these erosion channels. Beaman et al. proposed that high pressure at facet contact points may lead to pain at degenerated surfaces, that hypertrophy of the arthritic facet may also cause capsular stretch and pain and that this dual effect may play an important role in low back pain [108].
The objective in a study by Igarashi et al. was to quantify various inflammatory cytokines in facet joint tissue in surgical cases of stenosis and lumbar disc herniation. IL-1beta was detected in joint cartilage and synovium in both groups and TNF-alpha in the synovium in stenosis. IL-6 was high in joint cartilage and synovium in both groups. The concentration was significantly higher in stenosis than in LDH. The authors suggested that inflammatory cytokines in degenerated facet joints may have some relation to the cause of pain in degenerative lumbar disorders [109].
In a biomechanical study Yang and King reported on the effect of the eccentricity of the applied load on lumbar facet loads. They observed that as facet loads increased, the facets bottomed out on the laminae below, causing the facets to pivot and stretch the superior portion of the joint capsule [12]. El-bohy et al. included extensor muscle action to overcome moments due to eccentric loads which represented body weight and an external load acting 340 mm anterior to the center of the disc. Facet pressure was measured in all cases when muscle load was applied to counteract body weight [110] (Fig. 20.9). This pressure increased when more muscle force was applied to balance the externally applied flexion moment. When the anterior load was released suddenly, there was a large increase in facet pressure with a concomitant decrease in disc pressure [110].
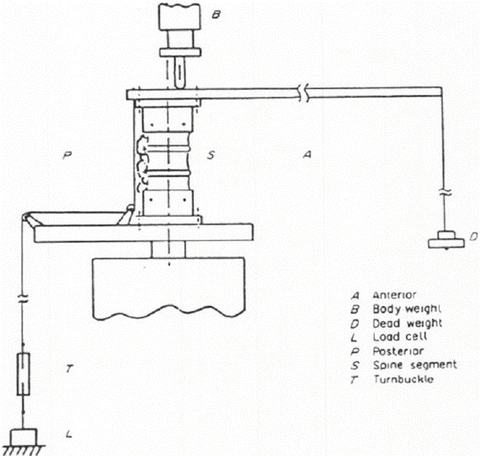
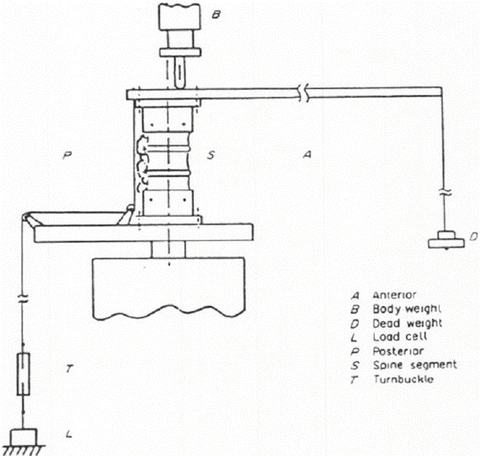
Fig. 20.9
Schematic diagram of the test set-up for measuring facet contact pressure in human cadaveric lumbar motion segments (Reproduced with permission from El-Bohy et al. [110])
El-Bohy et al. studied lumbar facet capsule strains with specimens loaded in flexion and extension. In extension, the facets bottomed out on the lamina below. This resulted in large capsular strains in the superior portion of the facet capsules which was quantified via video racking of targets placed on the capsule [110].
20.3.6.1 Facet Joint and Muscle Neurophysiology in Control Animals
The biomechanical studies of King, Yang and El-Bohy formed the biomechanical basis for a hypothesis that the facet joint capsule is a source of low back pain and that the pain may arise from large strains in the joint capsule that cause pain receptors to fire. To test this hypothesis, the following neuroanatomic and neurophysiologic studies were performed.
Yamashita et al. performed neurophysiologic studies of sensory neurons originating from the L5–L6 facet joint and surrounding muscle by recording from split L5 lumbar nerve roots in anesthetized New Zealand White rabbits [111, 112]. Thirty mechanosensitive units were identified at the facet joint and 27 others in the muscles and tendons near their insertion into the facet [112] (Fig. 20.10). Of the 30 units at the facet joint, 13 were in the capsule, 15 in the border regions between capsule and muscle or tendon, and two in the ligamentum fiavum (Fig. 20.10). Of the 30 units in the facet joint, two units had CVs <2.5 m/s (group IV), 17 units had CVs of 2.5–20 m/s (group III), and 11 units had CVs >20 m/s (group II). Nine units had thresholds >6.0 g, 17 units had thresholds <6.0 g, and four units were not examined. Eight units responded to joint movement caused by pulling on the isolated L5 lamina. Five of these were in the medial aspect of the facet joint. These units were most responsive to 1–2 mm of caudal-to-rostral stretch. The two units in the ligamentum fiavum were most responsive to ventral-to-dorsal stretch. Of the 27 units in muscle and tendon, 1 unit had a CV < 2.5 m/s (group IV), 4 units had CVs of 2.5–20 m/s (group III), and 22 units had CVs > 20 m/s (group II). One unit had a threshold >6.0 g and 26 units had thresholds <6.0 g. Thus, the facet joint contained a much higher proportion of high-threshold, low conduction velocity units than muscle. It is these units that are likely to serve as a pain function. These studies demonstrated that some units in the joint capsule were indeed responsive to stretch. However, the stretch was applied through a somewhat non-physiologic means (pulling on a surgically isolated joint) so the next step was to determine whether spine loading would cause capsular stretch that would cause facet joint units to fire.
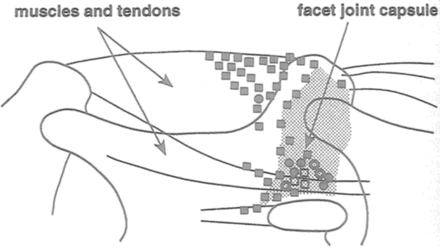
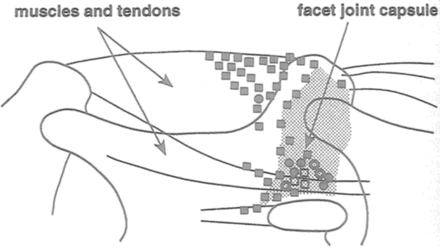
Fig. 20.10
Diagram localizing the receptive fields of the units that were identified at the facet joint and adjacent tissues in anesthetized New Zealand White rabbits. Square = units with thresholds of <6.0 g, circle = units with thresholds of >6.0 g. If the unit responded to stretch, the symbol is hollow (Reproduced with permission from Yamashita et al. [112])
20.3.6.2 Spine Loading Studies
Twenty-four in vitro experiments were performed in order to determine if facet joint receptors would respond to spine loading [113]. The abdominal aorta of the anesthetized New Zealand White rabbit was cannulated and perfused with Krebs solution oxygenated with 95 % O2, 5 % CO2, The lumbar spine was removed with bone rongeurs and placed in a chamber perfused with the same solution. Receptive fields were characterized and nerve recordings made for 2–3 h as the spine was loaded in tension and compression. A 100 lb load cell (Entran Devices ELF-100, Fairfield, NJ) recorded tensile and compressive loads. In these experiments a typical response was a vigorous multi-unit discharge during loading. During compressive loading the spine underwent dorsal-lateral bending that caused the facet joints to articulate along the joint plane, stretching the facet joint capsule. Loading excited both units with spontaneous activity, as well as units that were silent before loading was initiated. Three patterns were observed: (1) short duration bursts during onset or change in loading (phasic-type responses), (2) prolonged discharges that began during low levels of loads of 300–500 g (slowly adapting, low-threshold mechanoreceptors), and (3) prolonged discharges activated by higher loads of 3–5.5 kg (slowly adapting, high-threshold mechanoreceptors). It is this latter response which strongly suggested activation of pain fibers. Fourteen small myelinated or unmyelinated fibers (groups III and IV) responded around the facet joint. It was often not possible to track the response of these individual units to spine loading as this loading naturally elicited firing from many nerves.
20.3.6.3 The Effects of Facet Joint Inflammation on Nerve Activity
In both the in vivo and in vitro experiments described above, nerves typically stopped firing after the mechanical stimulus was removed or shortly thereafter, even if the stimulus was noxious. Thus, these results demonstrated that capsular stretch could cause the onset of facet pain but does not explain its persistence. It is likely that obvious tissue damage (i.e. tearing of muscle or capsule) would result in persistent discharge. The possible role of inflammatory mediators in facet joint pain was studied as a step in understanding the persistence of low back pain. These studies focused on neurogenic and non-neurogenic inflammatory mediators. Kaolin (a silica product) and carrageenan (a seaweed product) are commonly used to produce an acute tissue inflammation which results in a release of histamine, bradykinin and prostaglandins into extracellular tissue. These algesic (pain-producing) chemicals can excite and/or sensitize nerve endings [114–116].
Ozaktay et al. demonstrated the effects of inflammation in rabbit lumbar facet joint capsule and adjacent tissue. Type II carrageenan was injected into the receptive fields of mechanosensitive sensory neurons. Background discharge rate increased in two phases over a time period of 150 min: the first phase (0–30 min) and the second phase (45–150 min). Silent unit discharge rates increased in the first 15 min and persisted beyond 75 min. Histological examination revealed inflammation in carrageenan injected tissues. In normal saline injected controls there were no changes observed. The electrophysiological results showed that inflammation of the facet joint and deep back muscles caused (1) increases in multi-unit discharge rate, (2) sensitization to mechanical stimuli and (3) recruitment of previously silent units [117].
20.3.6.4 The Effects of Substance P on Unit Activity
The carrageenan studies demonstrated that tissue inflammation had a profound effect on the mechanical sensitivity and discharge rate of facet joint units. Another aspect of tissue inflammation is neurogenic inflammation and is the result of inflammatory chemicals released from the nerves themselves. One of the most studied neurogenic mediators is substance P, an eleven-amino-acid neuropeptide released from small nerve fibers. It has been shown to be released from nerve endings in the knee joint [118]. Substance P causes vasodilatation, plasma extravasation and release of histamine from mast cells [116]. These are important in the inflammatory cascade which can prolong pain. Substance P is also released at nerve synapses in the dorsal horn of the spinal cord to facilitate action potential transmission in spinal cord pain pathways [119, 116]. Substance P has been demonstrated in nerves of lumbar spinal tissues [78] and in small nerve fibers of the facet joint [120]. However, its effect on lumbar spinal tissue nerve activity had never been studied. In 15 rabbits a study was undertaken to examine how substance P affects the mechanosensitive afferent units identified in the lumbar facet joint and adjacent tissues of the rabbit [112]. Substance P was applied to the receptive fields of the units by means of microinjection and afferent activity of the units was recorded from dorsal root filaments. Changes of afferent discharge rates and von Frey thresholds were measured for 30 min. Most of the units (83.3 %) showed an increase in spontaneous discharge rate after the injection of 10 ug of substance P into the receptive field; 54.2 % of the units showed immediate onset and 29.2 % of the units showed slow onset of the excitation [112] (Fig. 20.11). One-third of the units showed decreased von Frey thresholds after the application of substance P (evidence of sensitization). Substance P had an excitatory effect on 81.8 % of the units with thresholds >5.0 g and conduction velocities <30 m/s that may serve as nociceptors, and 84.6 % of the units with thresholds <2.0 g which may serve as proprioceptors. These studies suggest that release of substance P may contribute to transmission of both nociceptive and proprioceptive sensations. Substance P may have had a direct effect on the nerve endings in paraspinal tissue, or acted indirectly through its influence on vasodilatation, plasma extravasation and histamine release [112].
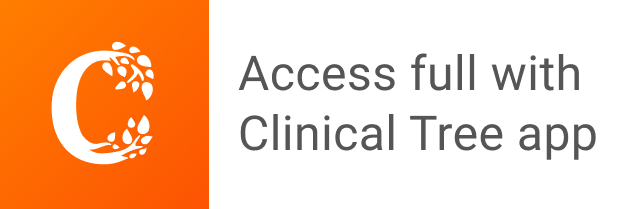