Fig. 4.1
The human cutaneous microvascular system. Three dimensional (3D) structure of the superficial microvascular system of human finger skin obtained by scanning electron microscopy (SEM) of corrosion casts [83]. On the palmer side of the finger (a), two rows of umbrella-shaped capillary loops follow the patterning of the fingerprint (see also Fig. 4.2). The capillary loop structures are ~100 μm high and have a dextrogyrate rotation, with a distance between each of ~70 μm (b). On the dorsal side, the capillary loops are randomly distributed (c) [Images reproduced with permission from [83]]

Fig. 4.2
The human microvascular fingerprint. Superimposition of the 3D structure of the superficial microvascular system (obtained by SEM of corrosion casts) (b) onto the original structure of the human finger (a, obtained earlier in the corrosion process) [83]. There is perfect correspondence between the alignment of the capillary loops and the fingerprint on the palmer side of the finger [Images reproduced with permission from [83]]
During heat stress, our skin contributes to body cooling by the generation and evaporation of sweat, and by dissipation of heat from the blood to the environment [6, 9, 10, 20]. Loss of heat from the skin vasculature is facilitated by the large cross-surface area of the vascular networks running parallel to the skin surface (e.g., Fig. 4.1) and by vasodilatation of skin blood vessels to increase blood flow. The structure and regulation of the cutaneous circulation differs between “hairy” skin (termed non-acral or non-glabrous skin), which covers most of the body surface and non-hairy skin (acral or glabrous), such as in the palmer aspects of the fingers, and in the nose, ears, palms, and plantar aspects of the feet and toes [72]. Glabrous skin contains a rich concentration of arteriovenous anastomoses (AVAs), which provide direct connections between arterioles and venules [6, 72, 96]. These structures allow blood flow to bypass the nutritional capillary loops, and so AVAs do not contribute to nutritional blood flow (Fig. 4.3). They are key thermoregulatory structures that can rapidly distribute high volumes of blood to the skin surface, enabling optimal regulation of core temperature [6]. Indeed, when there is need for heat conservation AVAs remain predominantly closed, whereas during heat elimination they are fully dilated and open [7]. In skin areas with AVAs, these structures enable blood flow to be rapidly altered by more than 100-fold. Three-dimensional analysis reveals remarkable heterogeneity of AVAs in the human finger, including direct connections (end-to-end, side-to-side, and end-to-side) and more complex structures [53, 69, 83] (Fig. 4.3). AVAs are located in both the lower and upper vascular plexuses [69, 83].


Fig. 4.3
AVAs in human finger skin. Corrosion casting demonstrates the heterogeneity of AVA structures in the cutaneous circulation of the human finger, with end-to-side (a), end-to-end (b), and more complex structures (c) [69, 83]. Within each image, A/a refers to arterioles and V refers to venules [Images reproduced with permission from [69, 83]]
In contrast to most organs, the skin circulation is innervated by two distinct types of sympathetic nerve fibers: a traditional sympathetic adrenergic innervation, which releases norepinephrine to cause vasoconstriction, and a sympathetic cholinergic innervation, which releases acetylcholine to cause vasodilatation. Although non-glabrous skin has sympathetic adrenergic constrictor and cholinergic dilator innervation, glabrous AVA-containing skin lacks influence from the cholinergic dilator innervation [72]. Furthermore, the sympathetic cholinergic vasodilator nerves do not regulate cutaneous blood flow under thermoneutral conditions, and are only activated during hyperthermia [20, 58]. This unusual sympathetic cholinergic innervation also innervates sweat glands and stimulates heat loss via increased production of sweat, the generation of which also requires increased blood flow [9, 10, 20]. The sympathetic adrenergic system provides basal constrictor activity to the skin circulation, particularly in the extremities, contributing to a relatively low skin blood flow even in thermoneutral environments [9, 10, 20]. Body cooling further increases cutaneous sympathetic adrenergic constrictor activity and reduces finger blood flow, whereas body heating inhibits the basal activity of these nerves and increases finger blood flow [9, 10]. In both thermoneutral and cool environments, the sympathetic constrictor outflow to cutaneous blood vessels in the hands and feet is much greater than that to more proximal skin areas including the forearm [9, 10].
AVAs are richly innervated by the sympathetic adrenergic system [6, 67, 72]. In a thermoneutral environment, AVAs display cycles of constriction and dilatation, which occur at a frequency of 2–3/min [6, 14, 27]. This cyclical vasomotion is synchronous between AVAs at different anatomical locations (e.g., hands and feet), and is thought to be mediated by synchronous bursts of sympathetic adrenergic nerve activity [6, 14, 27, 66, 80, 96] (Fig. 4.4). AVA-independent nutritional blood flow in both glabrous and non-glabrous skin does not display such cyclical fluctuations [27, 66, 67, 78, 80, 96]. Reflex changes in sympathetic vasoconstrictor activity in response to changes in environmental temperature have a dramatic effect on AVA blood flow: during whole body heating AVA vasomotion is reduced because the structures are continuously dilated and open, whereas during whole body cooling AVA vasomotion is reduced because the anastomoses are continually constricted and closed [14, 96]. Sympathetic vasoconstrictor activity appears to have a greater impact to restrict blood flow through AVAs compared to nutritional blood flow in glabrous and non-glabrous skin. Indeed, in contrast to blood flow in AVAs, nutritional blood flow in glabrous finger skin is not influenced by increased sympathetic activity during moderate body cooling [27]. This divergence is also evident when sympathetic activity is increased via non-thermosensitive mechanisms, causing reduced digital artery blood flow without affecting nutritional blood flow [78]. Likewise, blood flow to non-glabrous skin is affected less by whole body cooling and reflex cold-induced vasoconstriction than flow through AVAs in glabrous skin [80, 96]. Nutritional blood flow in the digits therefore appears to be protected from sympathetic constrictor activity [78]. Increased sensitivity of AVAs to sympathetic activation is also observed in response to local intra-arterial infusion of norepinephrine, which has a greater impact on reducing total digital blood flow and blood flow through AVAs compared to nutritional blood flow [27]. The increased influence of sympathetic activity on AVAs compared to nutritional blood flow may reflect increased expression of α-ARs, increased sympathetic innervation, and increased wall–lumen ratio of AVAs [27].


Fig. 4.4
AVA activity. Simultaneous measurement of blood flow velocity in digital arteries of the third fingers from the left (closed circles) and right hands (open circles) of a control individual when both hands were at 35 °C (upper panel) or after the right hand was cooled to 19 °C [7]. Note the remarkable synchrony of blood flow in the fingers at 35 °C, representing the synchronous burst of sympathetic constriction to AVAs in these anatomically distinct locations. During local cooling of the right hand, this vasomotion in the right finger was virtually abolished as a result of sustained closure of the AVAs [7]. Each symbol represents average velocity during 1 heart cycle (image adapted from [7])
In addition to reflex increases in sympathetic constrictor activity during body cooling, local skin exposure to cold causes direct constriction of the cutaneous circulation. In the human finger, local cold-induced constriction is caused by constriction of the AVAs as well as the more proximal arteries supplying the finger [7, 8]. During local cooling, the constrictor phase of AVA vasomotion is prolonged, and with more exaggerated cooling there is sustained closure of AVAs and vasomotion abruptly ceases [7, 8, 67] (Fig. 4.4). This constriction is not mediated by an increase in sympathetic outflow but by direct local effects of cooling on the AVAs. AVAs appear to be more sensitive to moderate local cooling than blood flow through non-AVA nutritional arterioles [7]. During prolonged exposure to local cooling (after 5–10 min), the cold-induced vasoconstriction can be temporarily curtailed with periods of vasodilatation (the “hunting reaction”), which is mediated predominantly by transient opening of the AVAs [8].
Contrary to local cooling, which mostly affects AVAs, local warming has a greater impact on nutritional blood flow. AVA vasomotion is not affected by local warming and the accompanying vasodilatation appears to be mediated predominantly by dilatation of arterioles supplying nutritional blood flow [6, 7]. In non-glabrous skin, which does not contain AVAs, local warming of the skin initiates an increase in blood flow that is mediated initially by activation of local sensory nerve fibers and the release of neuropeptide vasodilators including CGRP [58, 72]. Both glabrous and non-glabrous skin are richly innerved with these sensory nerve fibers [72], suggesting that this mechanism likely contributes to local heat-induced increases in nutritional blood flow at both sites. If local heating is maintained, then the prolonged increases in blood flow appear to be mediated by increased activity of NO [58, 72].
Mechanisms Regulating Blood Flow Responses During Cold Exposure
Much of what we know concerning mechanisms that contribute to cold-induced regulation of the cutaneous circulation was derived using preclinical or animal models of the human circulation. Some of these mechanisms have subsequently been confirmed in the human circulation. Therefore, in each section we consider preclinical models and how these translate into regulation of the human cutaneous circulation.
Modulation of Sympathetic and Adrenergic Constriction
Preclinical Models
Mammalian biological systems function optimally at warm temperatures, which is why our regulatory systems strive to maintain normal core body temperature. It is therefore surprising that a key aspect of that thermoregulation, the activity or constriction of cutaneous smooth muscle cells, actually increases during cold exposure. Indeed, in most blood vessels, moderate and severe reductions in temperature inhibit contractility and cause vasodilatation [100]. Cold-induced dilatation of cutaneous blood vessels can be observed when blood vessels are studied in the absence of stimulation or during constriction to numerous stimuli [100]. An important exception is cold exposure during constriction of cutaneous blood vessels to sympathetic adrenergic activation. Indeed, moderate cooling dramatically increases contraction to sympathetic nerve stimulation in cutaneous blood vessels, but inhibits the response in deep blood vessels [39, 42, 43, 101]. A similar trend was observed when responses to norepinephrine were assessed: cold exposure amplified the constriction in cutaneous blood vessels and inhibited the response in deep vessels [39, 42, 43]. This suggested that a key determinant of the unique cutaneous cold-induced constriction might be at the level of smooth muscle ARs. Although most blood vessels contain one class of α-ARs, the α1-ARs, cutaneous arteries and veins have a much higher expression and activity of α2-ARs on their smooth muscle cells [23, 37, 39, 42, 43]. Indeed, when released by sympathetic nerves in cutaneous blood vessels, norepinephrine causes vasoconstriction by preferentially activating α2-ARs [39, 41]. This increased activity of α2-ARs contributes to the unique cold-induced constriction of cutaneous blood vessels. Indeed, cooling powerfully amplifies constriction to stimulation of α2-ARs, but inhibits constriction caused by α1-ARs [39] (Fig. 4.5). Furthermore, inhibition or antagonism of α2-ARs (but not α1-ARs) prevented cold-induced amplification of norepinephrine-induced cutaneous constriction [39]. These experiments provided physiological rationale for increased α2-ARs activity in cutaneous blood vessels, enabling them to withstand direct cold-induced vasodilatation and instead respond with enhanced vasoconstriction (Fig. 4.6). α1-ARs also appear to have increased activity in cutaneous blood vessels (relative to deep blood vessels), which provides them with a buffer or reserve capacity to withstand the inhibitory effects of cold exposure on smooth muscle contractility [39, 41].



Fig. 4.5
Cooling increases α2-AR but reduces α1-AR contractile activity in cutaneous blood vessels. Upper panel: Transient cooling (from 37 to 24 °C) causes a dramatic increase in contractile responses to activation of smooth muscle α2-ARs by norepinephrine or the highly selective α2-AR agonists UK14,304 and BHT-920 [39]. Upward movement of the trace indicates contraction of an isolated canine saphenous vein, and the bar at the top indicates the temperature of the blood vessel [39]. Lower panel: in contrast to α2-ARs, contraction of the same blood vessels by the highly selective α1-AR agonist St 587 is virtually abolished when cooling is applied during the response. Contraction was assessed using a similar approach to the upper panel, but contraction is expressed as a % of the maximal response to norepinephrine, and presented as means ± SEM [39] [Images reproduced with permission from [39]]

Fig. 4.6
Schematic representation of sympathetic neurotransmission in human skin. In sympathetic nerves, norepinephrine (NE) is located in small storage vesicles. During Body Cooling, there is increased activation of sympathetic nerve fibers and exocytotic release of NE, which causes blood vessel constriction by stimulating predominantly α2-ARs located on smooth muscle cells. Norepinephrine also activates prejunctional α2-ARs on sympathetic nerves to inhibit release of neurotransmitters, including NE. Local cooling amplifies α2-AR constrictor activity, but inhibits smooth muscle constriction and α1-AR constrictor activity. Smooth muscle α-ARs can also be activated by circulating norepinephrine and epinephrine (Epi) released from the adrenal medulla (and other sympathetic nerves). The endothelium releases NO, which causes dilation of smooth muscle cells. Local cooling is thought to reduce this dilation, which would result in further amplification of α2-AR mediated constriction. Increased sympathetic nerve activity in response to Body Cooling not only reduces heat loss by initiating cutaneous vasoconstriction but also initiates heat production by activating thermogenesis in brown adipose tissue (BAT). Perivascular adipose tissue (PVAT) has morphological, genetic and proteomic similarity to BAT and may contribute to perivascular thermogenesis. Metabolic activity in neighboring skin and muscle cells (e.g., by releasing metabolic mediators such as adenosine) will dilate nutritional arterioles by inhibiting release of norepinephrine and by directly relaxing the smooth muscle. Solid arrows indicate activation, whereas hatched arrows reflect inhibition
α2-ARs comprise three distinct subtypes: α2A-ARs, α2B-ARs, and α2C-ARs [22, 23]. Research that defined the role of these receptor subtypes in responses to cold employed a mouse model of the cutaneous circulation (isolated tail arteries and arterioles) and cultured cells artificially expressing the receptors. Cutaneous smooth muscle cells express α2A-ARs and α2C-ARs, but only α2C-ARs are selectively involved in cold-induced vasoconstriction [22, 23, 25]. At warm temperatures, constriction caused by α2-AR stimulation was mediated predominantly by α2A-ARs with no evidence for involvement of α2C-ARs [23]. However, although inhibition of α2C-ARs had no effect on α2-AR constriction at warm temperatures, it powerfully inhibited α2-AR constriction at cold temperature and abolished cold-induced amplification and vasoconstriction in cutaneous arteries [23]. Antagonism of α2A-ARs inhibited constriction at both temperatures, but cold-induced amplification remained intact, suggesting α2A-ARs were active at both warm and cold temperatures, but were not modulated by temperature [23]. Therefore, α2A-ARs appear to be permissive for cold-induced amplification of the α2C-AR response, which may reflect an indirect or direct interaction between these different receptor species [22]. Temperature regulation of α2C-AR activity reflects an intriguing cold-induced translocation of these receptors from intracellular stores to the cell-surface where they are accessible to stimulation by norepinephrine [2, 3, 22, 54]. Although cutaneous α2-ARs were originally considered to be thermosensors, they are more accurately described as thermo-effectors and are responding to cold-induced signaling within the smooth muscle cells. The key thermosensor appears to be the mitochondria of smooth muscle cells, which on exposure to cold generate a rapid increase in production of reactive oxygen species (ROS) [3]. These signaling intermediaries subsequently activate a signaling pathway RhoA/Rho kinase (ROCK), which promotes cold-induced translocation of α2C-ARs to the cell surface [2, 3, 22, 54]. Indeed, direct activation of this pathway at warm temperatures also mobilizes α2C-ARs to the cell surface [55]. Components of this signaling pathway are also responsible for driving expression of α2C-ARs in cutaneous smooth muscle cells [22, 24, 55, 74], which suggests that the prolonged exposure of cutaneous blood vessels to reduced temperatures might increase expression of these powerful cold effectors.
Confirmation of these results was obtained in an in vivo model that analyzed regulation of blood flow to the glabrous region of the mouse paw [52]. In mice with drug-induced paralysis of sympathetic nerve fibers (tetrodotoxin), local cold exposure caused vasoconstriction that was mediated by activation of α2C-ARs [52]. This reflected cold-induced amplification of vasoconstriction to circulating catecholamines (epinephrine, norepinephrine released from the adrenal medulla) [52]. Indeed, local cooling amplified constriction to activation of α2-ARs (intra-arterial clonidine) but not α1-ARs (phenylephrine). Furthermore, selective inhibition of α2C-ARs did not affect α2-AR constriction at normal temperatures, but abolished the cold-induced increase in constriction [52]. These results also demonstrate that cold-induced modulation of α2C-ARs can amplify responses to circulating catecholamines and does not require functioning sympathetic nerves.
Defining the role of smooth muscle α2-ARs (and α2C-ARs) during constriction to sympathetic nerve stimulation is more challenging because of prejunctional α2-ARs, which act to reduce neurotransmitter release from the nerve fibers (Fig. 4.6). Indeed, in non-cutaneous blood vessels, which are dominated by smooth muscle α1-ARs, α2-AR stimulation causes marked vasodilatation during sympathetic nerve activity by inhibiting the release of norepinephrine [77]. α2-AR inhibition or antagonism has the opposite effect, increasing vasoconstriction to sympathetic stimulation [77]. In cutaneous blood vessels, which are dominated by smooth muscle α2-ARs, α2-AR inhibition reduces sympathetic constriction, but this effect is diminished because of increased release of norepinephrine and subsequent activation of smooth muscle α1-ARs [41]. Prejunctional α2-ARs comprise both α2A-ARs and α2C-ARs, and in contrast to smooth muscle, the neuronal α2C-ARs are already localized to the cell surface [22]. Although α2-AR blockade abolished cold-induced amplification of constriction to norepinephrine, only combined blockade of α2-ARs and the constrictor activity of ATP (another transmitter released by sympathetic nerves) was able to prevent cold-induced amplification of constriction to sympathetic nerve stimulation [39, 45]. This likely reflects an increase in ATP release following inhibition of prejunctional α2-ARs, and a cold-induced increase in smooth muscle contraction to ATP [45].
The Human Circulation
As in preclinical models, the smooth muscle cells of human cutaneous blood vessels have increased expression and activity of α2-ARs compared to deeper blood vessels [25, 38, 40]. Indeed, the constrictor activity of α2-ARs increases markedly on going from proximal to distal arteries in human limb arteries, with powerful constrictor activity in small digital arteries [38]. In contrast, the activity of α1-ARs remains fairly constant [38].
Exposure to moderate whole body cooling caused a dramatic reduction in finger blood flow that was completely prevented by selective inhibition of α2-ARs (yohimbine) but not significantly affected by selective inhibition of α1-ARs (prazosin) [29]. This constriction is mediated by a reflex increase in sympathetic nerve activity and reduced blood flow through AVAs. Therefore, the results suggest that the smooth muscle of AVAs in the human finger are richly endowed with α2-ARs and that sympathetic vasoconstriction of AVAs, including in response to whole body cooling, is mediated preferentially if not exclusively by α2-ARs (Fig. 4.7). During this reflex increase in sympathetic activity, inhibition of α1-ARs or α2-ARs did not significantly affect nutritional blood flow in the finger, confirming the relative protection of finger nutritional blood flow from sympathetic responses [29].


Fig. 4.7
Schematic representation of the cutaneous vascular system in human glabrous skin. The arterial supply system branches to an arteriovenous anastomosis (AVA) and also to nutritional capillary loops. In response to Body Cooling, there is increased activity of sympathetic nerves, which release norepinephrine to cause constriction predominantly of the AVA structures by activating α2-ARs located on the smooth muscle cells. AVAs appear to have increased activity of this constrictor mechanism, whereas nutritional blood flow through capillary loops is protected from sympathetic constriction and is therefore maintained. Local cooling amplifies sympathetic α2-AR dependent constriction of AVAs
Intra-arterial infusion of α1-AR or α2-AR agonists causes profound vasoconstriction of human finger blood flow [29, 48]. Under conditions of body heating (to reduce cutaneous sympathetic nerve activity), local cooling amplified constriction evoked by activation of α2-ARs whereas it inhibited constriction to α1-AR stimulation [48]. In studies restricted to non-glabrous skin, mild local cooling causes rapid constriction that was markedly reduced by selective inhibition of α2-ARs and only slightly reduced by inhibition of α1-ARs [35]. Acute cold-induced amplification of α2-AR constriction in non-glabrous skin is also inhibited by blocking ROCK, which would be consistent with cold-induced mobilization of α2C-ARs [57, 95].
Therefore, results from preclinical models and clinical studies demonstrate that α2-ARs located on the smooth muscle of cutaneous blood vessels represent a key mechanism for initiating vasoconstriction in response to cold exposure (Figs. 4.6 and 4.7). During body cooling, the resulting reflex sympathetic vasoconstriction in human fingers is mediated by α2-ARs, confirming the preferential activation of these receptors by nerve-released norepinephrine in cutaneous blood vessels. Furthermore, the increased responsiveness of α2-ARs at cool temperatures provides a mechanism whereby local cooling can initiate constriction and amplify the response to sympathetic stimulation. Because of the increased responsiveness of AVAs to vasoconstriction by sympathetic stimulation, exogenous norepinephrine and local cooling, the activity of smooth muscle α2-ARs is likely to be increased in AVA compared to nutritional blood vessels (Fig. 4.7).
Secondary Vasomotor Mechanisms
In non-glabrous skin, although acute local cooling stimulates vasoconstriction that is mediated by α2-ARs [35], more prolonged cooling (e.g., 40 min [95]) causes constriction that is mostly resistant to inhibition of α1-AR or α2-ARs [35, 95]. However, both responses are mediated by ROCK [95]. Therefore, local cooling likely activates Rho/ROCK signaling, as demonstrated in preclinical models, which initiates cold-induced vasoconstriction by amplifying smooth muscle α2C-ARs [2] and then sustains constriction by α-AR independent mechanism(s) [57, 95]. Indeed, Rho/ROCK plays a central role in regulating contraction of smooth muscle cells by increasing the sensitivity of the contractile process to activator calcium ions [2, 95]. Vasoconstriction resulting from this calcium sensitization can be observed in cutaneous blood vessels during cold exposure [2]. It is not yet known if the cold-induced increase in ROS and Rho/ROCK signaling observed in smooth muscle cells also occurs in endothelial cells. If so, Rho/ROCK signaling can inhibit the production of NO through multiple mechanisms [107], whereas ROS can inactivate NO [15, 79]. Although the underlying mechanisms have not been defined, cold exposure inhibits NO-mediated vasodilatation [58, 72] (Fig. 4.6).
Transient Receptor Potential (TRP) Channels
Our perception or ability to sense temperature is mediated by cutaneous thermosensitive sensory neurons. Distinct systems and mechanisms are thought to mediate the sensation of innocuous (15–30 °C) and noxious cool (<15 °C), as well as innocuous and noxious warm temperatures [70, 71]. A key mediator in cold-sensation is TRP melastatin 8 (TRPM8) channels, which respond to innocuous cold temperatures (threshold activation at 22–27 °C) and may also contribute to the sensation of noxious cold [1, 70, 71]. TRPM8 channels can be activated by menthol, which shifts the thermosensitivity of the channels enabling them to be active at warmer temperatures, and is responsible for menthol’s pleasantly cool sensation [71]. How TRPM8 channels respond to cold temperatures has not been defined [70, 71]. Sensory nerve endings that express TRPM8 extend to multiple termination zones in glabrous and non-glabrous skin providing an ideal location to detect and respond to decreases in ambient temperature [33, 91]. However, they are not localized to cutaneous blood vessels and any TRPM8 expression in vascular structures is less than that of sensory neurons [33, 91]. Emerging evidence suggests that cold-induced activation of TRPM8 on cutaneous sensory nerve endings contributes to thermoregulation.
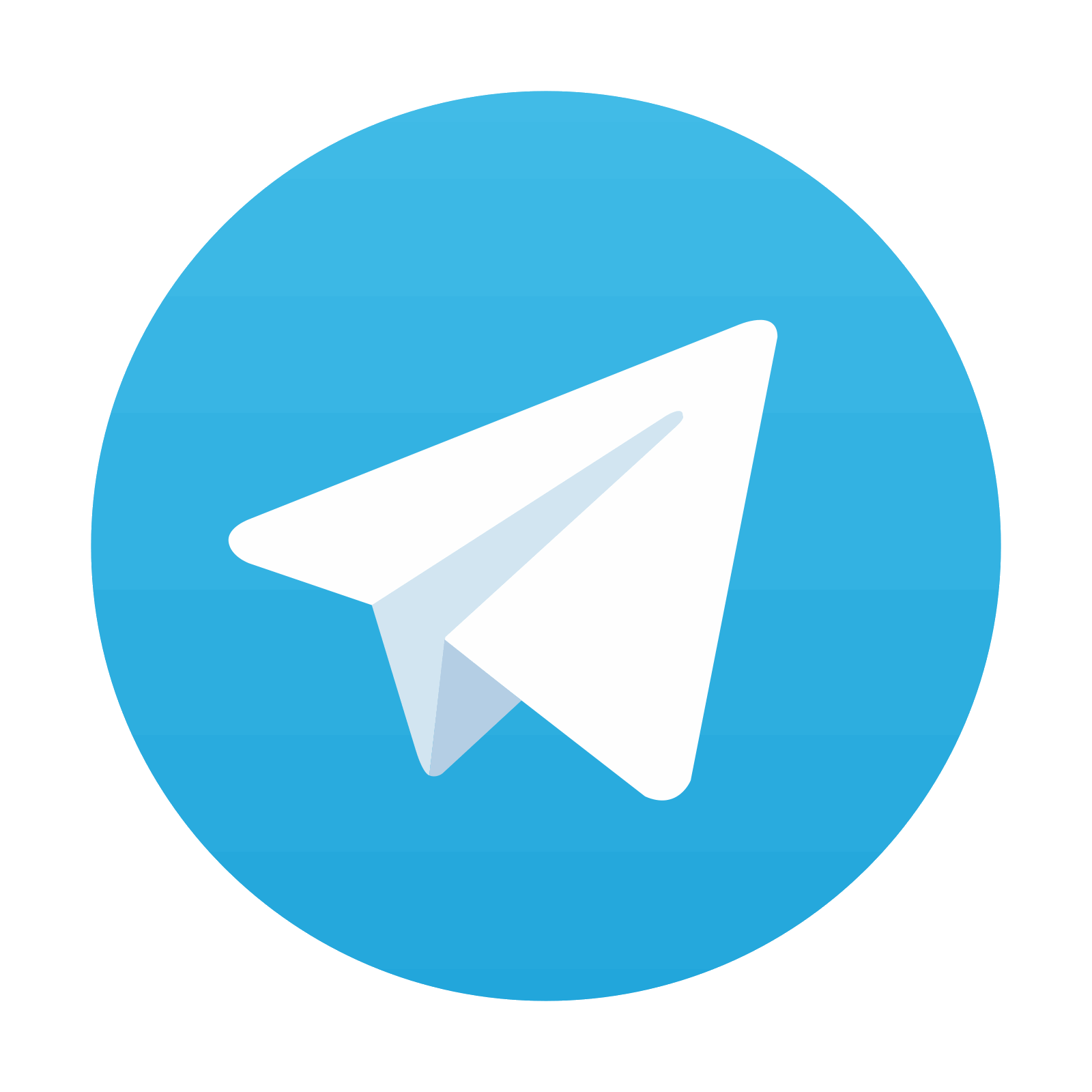
Stay updated, free articles. Join our Telegram channel
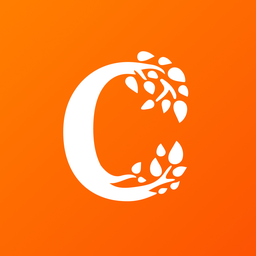
Full access? Get Clinical Tree
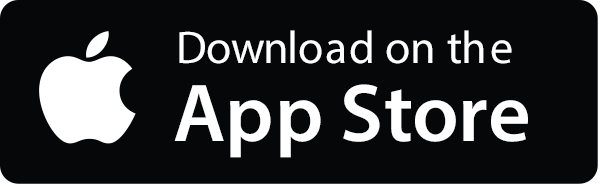
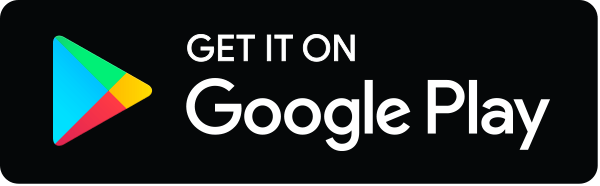