Fig. 12.1
Histologic cross section of a normal knee joint (Courtesy of Edward DiCarlo, MD, Hospital for Special Surgery, New York, NY)
Under physiological conditions, the subchondral bone adapts its structural and functional properties through a highly regulated cellular process involving distinct bone cell populations. The remodeling process involves the recruitment of myeloid lineage cells to the bone surface followed by differentiation of these cells into osteoclasts that are uniquely adapted to removal of the mineralized bone matrix. Multiple lines of evidence have established that the osteoclast is required for resorption of the bone under physiological conditions and also is the principal cell type that mediates bone resorption in pathological conditions [3–5]. The phase of bone resorption is followed by a reversal phase in which the resorbed bone surface is populated by bone-forming osteoblasts that replace the resorbed bone. The remodeling process occurs throughout postnatal life and provides a cellular system for adapting the bone to biomechanical influences, repairing damage to the bone matrix, and, under certain conditions, releasing calcium for maintenance of mineral ion homeostasis [6]. In addition to the osteoclast and osteoblast, there is a third cell type in the bone, the osteocyte [7, 8]. Osteocytes are embedded within the bone matrix where they form an interconnected network with each other and with the cells on the bone surface. They play a critical role in regulating bone remodeling in response to local soluble mediators and systemic hormones and importantly are the principal regulator of the response of the bone to alterations in mechanical loading [9]. The unique capacity of the bone to adapt to its local mechanical environment is embodied in Wolff’s hypothesis that states that the distribution and material properties of the bone are determined by the magnitude and direction of the applied load [10]. In this paradigm, the presence of increased bone volume is a reflection of increased load transfer and decreases in bone mass conversely reflect a relative decrease in the local loading history. The observed changes in the subchondral bone that accompany the adverse effects of excessive loading and injury will be described in the subsequent sections.
Bone Pathology in OA
Anatomic and histopathological studies have provided a comprehensive overview of the characteristic periarticular changes in human subjects with established OA. The changes include the presence of increased cortical plate thickness, decreased subchondral trabecular bone mass with localized regions of increased horizontal trabeculae, the presence of bone cysts, flattening and deformation of the subchondral articular contour, and osteophyte formation at the joint margins [1, 11–15]. The term “attrition” has been used to describe the flattening and change in the subchondral articular contour and reflects the influence of local biomechanical influences on bone remodeling [16–18].
In addition to the role of mechanical factors, bone remodeling also may be initiated at sites of local bone damage, which results from mechanical loading that is sufficient to produce disruption of the integrity of the skeletal architecture at a microscopic level, so-called microdamage. Damage of this type may occur with a distinct episode of excessive loading associated with joint injury or may occur in the context of repetitive loading in an individual engaged in repetitive physical activity. This form of microdamage is associated with the appearance of microcracks in the bone architecture [1, 10, 19, 20] and is distinct from traumatic bone injuries associated with fractures that disrupt the gross bone architecture. The process of the so-called targeted remodeling provides a cellular mechanism for repairing the focal bone damage but under certain conditions may contribute to the formation of bone cysts that represent one of the radiographic and anatomic hallmarks of OA [2]. As will be discussed, targeted remodeling associated with bone microdamage likely accounts for the bone marrow lesions observed with MRI in patients with established OA.
Although not well visualized using standard radiographic techniques, the zone of calcified cartilage also undergoes marked alterations in its cellular composition and organization during the evolution of the osteoarthritic process. These changes include expansion of the calcified cartilage and advancement and replacement of the matrix of the overlying articular cartilage associated with duplication of the tidemark [1, 11, 19]. This expansion of the calcified cartilage is associated with the penetration by vascular elements that extend from the subchondral bone and adjacent marrow spaces recapitulating the vascular invasion of the growth plate that occurs during the development and growth of long bones [21–24]. Figure 12.2 depicts the histopathological features of the periarticular bone with expansion of the zone of calcified cartilage, tidemark duplication, and vascular invasion of the subchondral cortical bone.
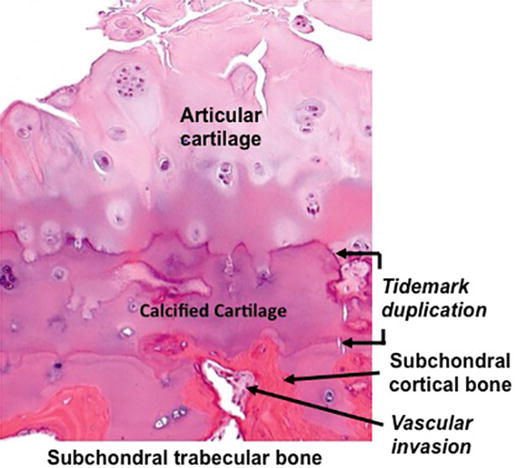
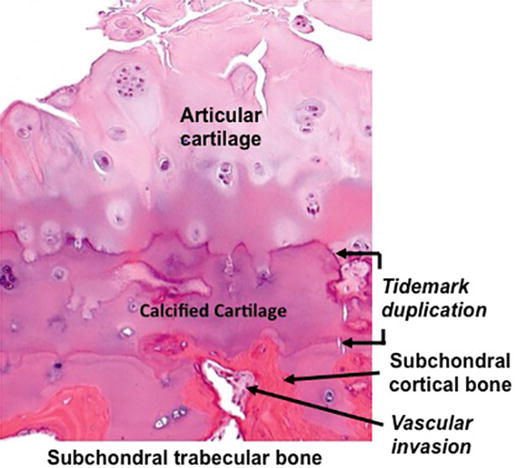
Fig. 12.2
Subchondral bone changes associated with advanced osteoarthritis. There is advancement of the calcified cartilage into the lower zones of the articular cartilage with duplication of the tidemark and vascular invasion of the subchondral cortical bone (Courtesy of Edward DiCarlo, MD, Hospital for Special Surgery, New York, NY)
The mechanical properties of the subchondral bone are influenced by the organization and composition of the organic bone matrix and the mineral content, which are highly dependent on rate of bone remodeling [25–27]. In physiologic remodeling, bone formation is initiated by the deposition of the organic bone matrix (osteoid), which undergoes rapid mineralization. After this initial phase there is a late phase of mineral accretion, which markedly influences the material properties of the bone matrix. In states of high bone turnover, the “late” phase of mineral accretion is attenuated by the rapid remodeling process, leading to a state of relative hypomineralization. This is associated with a reduction in the elasticity modulus of the bone that is more easily deformed under load. In contrast, in low bone turnover states, the continued deposition of mineral leads to an increase in the elastic modulus, and the bone becomes resistant to deformation and more “brittle.” During the progression of OA, marked changes occur in the rate and extent of remodeling in the subchondral bone, and these changes in bone turnover affect the state of mineralization and modify the capacity of the bone to deform under load, which alters the susceptibility to both micro- and macrodamage [11, 25, 28].
Post-Traumatic OA: Response of the Bone to Injury
Epidemiological studies have helped to identify the role of joint injury in the pathogenesis of OA. Although it is challenging to make precise estimates, long-term studies from patients with knee ligament and meniscus injuries demonstrate a tenfold risk of OA compared to those without injuries, and the numbers are much higher for individuals who have sustained intra-articular fractures [29]. The study of individuals with OA associated with a specific joint injury has been informative in establishing the evolution of the sequential joint pathology. A retrospective analysis in patients with anterior cruciate ligament (ACL) rupture reveals that 60–90 % of the patients exhibit radiographic features of OA within 10–15 years, including both cartilage and bone pathologies [30–32]. When the ACL injury is accompanied by injuries to the collateral ligament and menisci, there is evidence of more rapid appearance and progression of the OA changes, indicating that the magnitude of the injury and the resulting alterations in joint mechanics play a contributory role to the more unfavorable natural history [31, 32]. Buckland-Wright and coworkers [33] performed a cross-sectional study of patients with anterior cruciate ligament rupture to define the sequence of periarticular bone changes. Their analysis revealed the development of progressive thickening of the subchondral horizontal trabeculae within three to four years after the injury. They detected osteophytes in approximately 50 % of the injured knees by the third year but did not observe a change in joint space width or cortical plate thickness. They noted that these findings contrast with the changes in the subchondral bone observed in patients with knee OA not associated with a discrete injury in which the increased thickness in the subchondral bone plate appears to antedate the alterations in the trabecular bone. Although they speculated that the differential patterns could be related to differences in the biomechanical and adaptive responses, they acknowledged that differences in the two groups could reflect the relatively short duration of the study in the patients with joint injury. It would be predicted that with the passage of time, the subchondral and periarticular bone changes in patients with or without traumatic joint injuries would exhibit similar features, since factors such as joint instability and mechanical overload are major contributory factors to OA progression in both conditions.
Further insights into the natural history of post-traumatic periarticular bone changes have been provided through the study of animal models in which it is possible to control the many variables that impact on the evolution of the bone changes. Multiple species have been investigated in these studies and a variety of models exist based on the site and type of the tissue injury or disruption. However, the reported findings with respect to the subchondral bone are inconsistent and in some instances conflicting results have been obtained even among the studies using the same animal model. Nevertheless, these studies have been informative, and it is possible to draw several general conclusions from the results. Kuroki and Cook [34] utilized three different canine models of OA to define the evolution of the subchondral bone changes after joint injury. OA was initiated in one knee of mongrel dogs either by ACL transection, medial femoral grove creation, or medial meniscal release, and the knee joints were examined 12 weeks after surgery by histology and histomorphometry. A matching group of dogs underwent sham surgery and the non-operated knee in each of the groups served as an additional control. OA articular pathology assessed using the Mankin scores was present in all of the treatment groups. A significant decrease in subchondral bone plate thickness, trabecular thickness, and trabecular bone volume fraction compared to the sham-operated controls was observed in the ACL transection group. These findings were similar to those reported by others using this canine model [35–37]. Thinning of the subchondral plate was also observed in the groove model. In contrast, they observed significant thickening of the subchondral plate in the medial meniscus release group. A similar increase in the subchondral plate thickness after medial meniscectomy has been reported in a rabbit [38] and mouse model [39]. In the canine model study by Kuroki and Cook [34], the knee joints from the dogs in the meniscectomy group showed the most severe cartilage damage compared to the other groups, and the authors speculated that, although there may have been an early decrease in cortical plate thickness, as the OA progressed, the subchondral plate underwent a progressive adaptive increase in thickness. An important observation in these studies is that there was a good correlation between the changes in cortical plate thickness and the cartilage pathology in all of the models, which supports multiple other lines of evidence that both the bone and cartilage undergo cellular and morphological adaptation to local mechanical factors associated with the alterations in joint mechanics and loading induced by the initial injury.
A general feature of many of the animal models of PTOA is that they produce a significant injury to the joint tissues related to the surgical procedure. This may lead to alterations in the subsequent pattern of weight bearing and activity that could have a major influence on the subchondral bone adaptation. In the studies by Kuroki and Cook [34], the dogs that had undergone ACL transection still exhibited evidence of lameness, and they observed that the lameness scores correlated with the decreased bone volume and subchondral cortical bone thickness. In previous studies by the authors [40], they observed that lameness scores correlated with force plate patterns of loading, indicating the importance of the joint surgery on the subsequent pattern of physical activity and joint loading, which would be predicted to have significant effects on bone adaptation.
Lacourt et al. [41] utilized a model of repetitive impact-induced injury to examine the relationship between joint trauma and OA pathogenesis. They conducted a postmortem exam of the third carpal cuboidal bone in a group of fifteen racehorses that were exposed to repetitive trauma-induced OA at this skeletal site. Multiple analytic techniques revealed the presence of subchondral bone pits associated with articular cartilage damage manifested by fibrillation, fissuring, erosion, ulceration, and loss of proteoglycan. Histological analysis revealed extensive microcracks in the calcified cartilage and in localized regions of the subchondral bone plate. There were extensive areas of vertically oriented resorptive remodeling in the cancellous bone beneath many of the pits. A micro-CT analysis revealed reduced bone density, bone volume, and trabecular thickness with greater trabecular spacing in these regions. The authors speculated that the repetitive compressive forces and cumulative microdamage were responsible for the induction of remodeling changes that produced the alterations in bone architecture.
The presence of active bone remodeling topographically associated with microdamage in the calcified cartilage has been described in both human and equine OA [42–45]. These changes are often accompanied by advancement of the zone of calcified cartilage into the overlying hyaline articular cartilage and duplication of the tidemark [19, 20, 46, 47] (Fig. 12.2). Histologic examination of this zone reveals the presence of penetration of the calcified cartilage by vascular elements and formation of new calcified cartilage and bone, recapitulating the morphological features of the growth plate. Walsh and coworkers [22–24, 48] have examined the osteochondral junction in patients undergoing arthroplasty for end-stage OA using immunostaining techniques and noted the presence of sensory nerve fibers expressing nerve growth factor in the vascular channels associated with osteochondral angiogenesis. They hypothesized that the sensory fibers in these regions could be a potential source of symptomatic pain. The regions of vascular invasion also were associated with localized replacement of the bone marrow by fibrovascular tissue with cells expressing vascular endothelial factor (VEGF). VEGF also was detected in chondrocytes that were in proximity to the new blood vessels, and the authors speculated that the VEGF could provide signals for recruitment of the vascular elements.
An additional feature of OA is the detection of so-called bone marrow edema in the subchondral bone most often associated with sites of OA cartilage pathology. The bone marrow edema signal is characterized by increased signal intensity using fluid-sensitive magnetic resonance sequences [49, 50] (Fig. 12.3). The histologic examination of the anatomic sites corresponding to regions of bone marrow lesions has revealed the presence of regions of localized marrow fibrosis and fat necrosis associated with microfractures of the trabecular bone. The presence of microfractures and localized bone remodeling is consistent with localized activation of bone repair processes that accompany targeted bone remodeling [1, 20], and these findings indicate that the MRI signals are not generated by actual “edema” but rather by the replacement of the hematopoietic marrow with the reactive bone remodeling and repair process [51, 52]. Longitudinal studies indicate that bone marrow lesions may come and go, but importantly, their presence has been shown to correlate with the severity of joint pain and with progression of OA cartilage and bone pathology [50, 53–57]. The correspondence of the sites of bone marrow lesions with regions of bone and cartilage damage supports the concept that mechanical factors, including local traumatic bone injury, are responsible for the pathogenesis of the subchondral bone marrow changes. Further support for this concept is provided by the observation that bone cysts associated with OA frequently develop in the focal areas of bone damage and necrosis [58, 59].
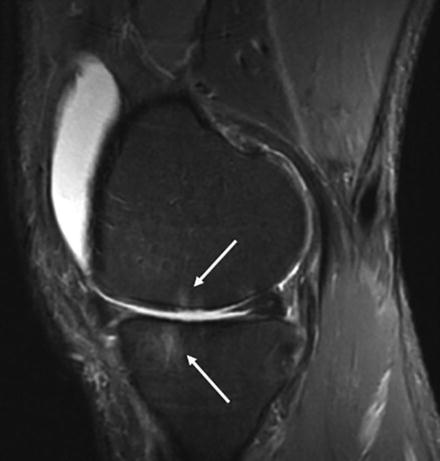
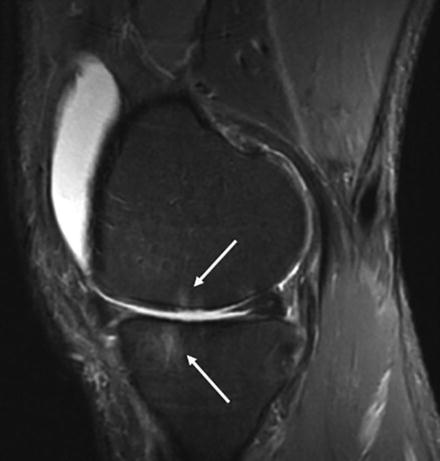
Fig. 12.3
MRI using fat-suppressed T2-weighted fast spin-echo (STIR) sequence demonstrating the presence of bone marrow lesions in the distal femur and proximal tibia in a patient with OA (Courtesy of Hollis Potter, MD, Hospital for Special Surgery, New York, NY)
An additional condition associated with the presence on MRI of bone marrow lesions has been described in patients with so-called bone bruises or contusions [60]. These lesions demonstrate similar characteristic to the bone marrow lesions detected in patients with OA but differ in their natural history and histological and pathological features. Characteristically, they are detected after an acute joint injury and have been described at multiple different skeletal sites, although the knee has been the most studied site. An analysis of the anatomic and histologic features of the tissue pathology associated with these lesions reveals the presence of bleeding, infraction, and edema related to true microscopic compression fractures of the cancellous bone. Mink and Deutsch [61] have classified bone bruises of the knee into four types: bone bruises, stress fractures, femoral and tibia fractures, and osteochondral fractures. According to the authors, the subtypes can be distinguished by their distinct patterns on MRI sequences and their anatomic localization. Bohndorf [62] proposed a classification of subchondral lesions in the knee based on the presence or absence of disrupted articular cartilage and separated these subtypes into “classic bone bruises” and subchondral impaction fractures. In general, the contusion patterns and location are a reflection of the mechanisms and site of the injury. According to this model, the pivot shift injury associated with anterior cruciate ligament disruption results in bone marrow lesions localized to the posterior aspect of the lateral tibial plateau and the midportion of the lateral femoral condyle [61], which correspond to the sites of acute impact loading during the injury. Roemer and Bohndorf [63] examined the long-term outcomes in a series of patients with acute traumatic knee injuries using MRI. They found a prevalence of bone bruising of 72 % in 176 patients, 25 of whom had isolated bone bruising in the absence of detectible subchondral fracture or overt cartilage disruption. 49 patients were evaluated with MRI after a minimum of 2 years, and they concluded that in the absence of a fracture, acute bone bruising vanishes in a majority of patients. The natural history in patients with subchondral fractures or osteochondral lesions may be much less favorable, and in these patients the presence of the bone bruise and the accompanying bone and cartilage pathology may be associated with a more rapid appearance and progression of periarticular and joint pathology.
Osteophytes are an additional characteristic radiographic feature of OA. Numerous lines of investigation implicate local biomechanical factors in their pathogenesis. Their location at the joint margins and their frequent association with the presence of joint instability has suggested that they may serve to stabilize the joint rather than contributing to joint dysfunction and OA progression [64, 65]. This conclusion is supported by the observations of Pottenger et al., who noted that removal of medial and lateral osteophytes from the knee joint increased joint instability [66]. Further evidence suggesting that osteophytes may not play a primary contributory role to OA progression has been provided by the studies of Felson and coworkers [67] who examined the relationship between osteophyte size and the risk for structural progression of knee OA. They found that the presence of large osteophytes did not appear to affect the risk for OA structural progression and speculated that the relationship between osteophytes and OA progression was more likely attributable to the presence of malalignment that contributed to both the formation of osteophytes and the progression of OA.
Studies in animal models of OA have provided insights into the mechanisms involved in the formation of osteophytes. Histologic analysis suggests that osteophytes are initiated by the proliferation of periosteal cells at the joint margins. As the process proceeds, these cells undergo differentiation into chondrocytes, which hypertrophy and through the process of endochondral ossification create an enlarging skeletal outgrowth at the joint margin [65]. Growth factors, including transforming growth factor β and bone morphogenic protein-2, have been implicated in the formation and growth of the osteophytes [68, 69]. This conclusion is supported by the observations that intra-articular injection of these growth factors into joints in animal models induces the formation of osteophytes and, conversely, inhibition of their activity or interference with their signal pathways impairs osteophyte formation [70–74]. Although local mechanical factors are believed to be responsible for induction of the growth factors, the mechanisms involved in this process are not well understood.
In addition to the influence of mechanical factors on the initiation and progression of bone changes in OA, biological processes may also influence the remodeling and adaptation of the periarticular bone. These effects may be mediated by products derived from other joint tissues, including the synovium, menisci, adipose tissues, and cartilage. Recent studies have shown that soluble products can be directly exchanged between the subchondral bone and cartilage via a process of diffusion, providing a mechanism by which these two tissues can interact with each other to influence the activities of their resident cell populations [75–77]. The vascular invasion of the calcified cartilage and the advancement of the tidemark that are associated with OA represent biological processes that may result from these types of interactions [22–24, 48].
In summary, traumatic injury to the joint represents a major risk factor for the development of OA. The type and magnitude of the injuries may be highly variable and include a spectrum of alterations ranging from disruption of supporting ligamentous structures to overt intra-articular fractures. The progressive alterations in the structural and functional properties of the periarticular bone are dependent not only on the direct effects of the injury on the bone tissues but may result from the adverse effects of the initial injury on joint mechanics. This in turn produces alterations in the local biomechanical environment that modulate the activities and function of resident bone cells leading to progressive alterations in their synthetic and reparative activities (Fig. 12.4). Therapies to reduce the risk and incidence of PTOA must therefore be directed not only at targeting the bone cells but, importantly, at restoring physiological joint structure and function after the injury. This includes the institution of preventive programs that can reduce the risk of the initial injury. In the absence of gross anatomic disruption of the bone structure associated with fractures, the periarticular bone changes that occur after joint injury are not unique to PTOA and recapitulate the skeletal changes that are characteristic of other etiological forms of OA. An understanding of the pathophysiological processes associated with the periarticular changes that occur in OA provides a rationale framework for developing therapeutic interventions that have the potential to modify the natural history of the bone changes. Although therapies that specifically modify bone cell activity and remodeling have shown favorable effects on the progression of OA in animal models, these treatment interventions have not been successful in human trials. In part this lack of success can be attributed to the marked heterogeneity of the patient populations with OA and the complex processes associated with skeletal remodeling. Future studies will therefore require more rigorous classification and characterization of the stage of OA progression and the unique and specific etiologic mechanisms responsible for the bone and joint pathology.
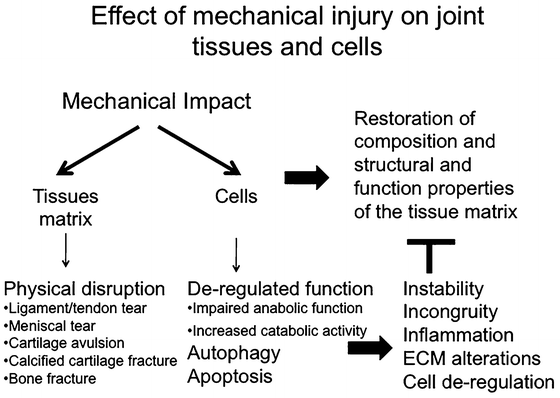
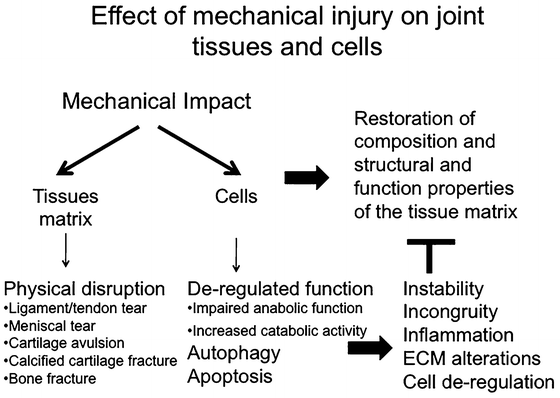
Fig. 12.4
Effects of acute traumatic mechanical injury to joint tissues. The magnitude of the energy of the impact determines the pattern and extent of the joint tissue injury and outcomes in terms of joint homeostasis. High-energy impact acutely disrupts the structural integrity of the joint tissues leading to irreversible alterations in joint stability and mechanics. Low-energy impact may not directly disrupt the integrity of the extracellular matrices of the joint tissues but may instead induce a cascade of events that modulate the activities and function of the resident cell types. This can initiate progressive alterations in their synthetic and reparative activities that modify the material properties of the tissues that they populate, leading to deterioration in the structural and functional properties of the tissues
References
1.
Burr DB. Anatomy and physiology of the mineralized tissues: role in the pathogenesis of osteoarthrosis. Osteoarthritis Cartilage. 2004;12 Suppl 1:20–30.CrossRef
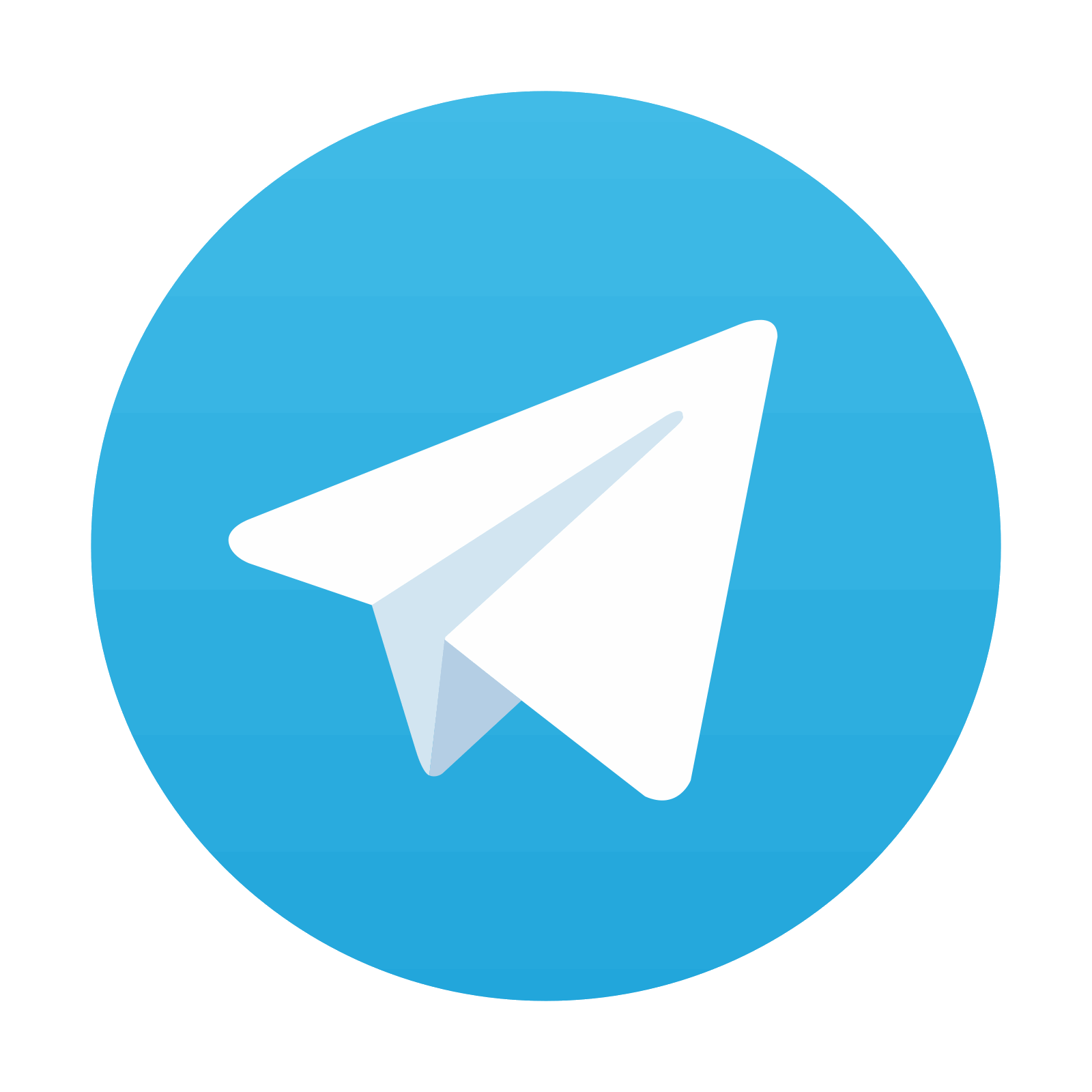
Stay updated, free articles. Join our Telegram channel
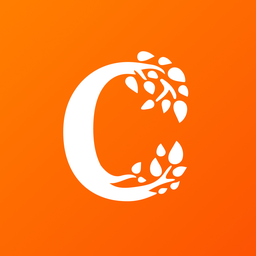
Full access? Get Clinical Tree
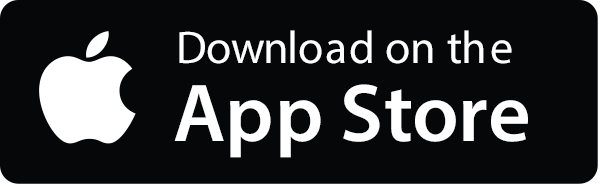
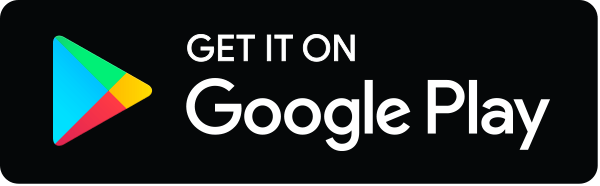