Mechanics play a role in the initiation and progression of osteoarthritis. However, our understanding of which mechanical parameters are most important, and what their impact is on the disease, is limited by the challenge of measuring the most important mechanical quantities in living subjects. Consequently, comprehensive statements cannot be made about how mechanics should be modified to prevent, slow or arrest osteoarthritis. Our current understanding is based largely on studies of deviations from normal mechanics caused by malalignment, injury, and deformity. Some treatments for osteoarthritis focus on correcting mechanics, but there appears to be scope for more mechanically based interventions.
Key points
- •
Mechanics play a role in the initiation, progression and successful treatment of osteoarthritis. Many of the hypotheses proposed to explain why osteoarthritis begins and progresses center on mechanics.
- •
Not enough is known about which specific mechanical parameters are most important, and what their impact is on the disease process, so comprehensive statements cannot be made about how mechanics should be modified to prevent, slow or arrest osteoarthritis.
- •
Our understanding of the role of mechanics in osteoarthritis is limited by the challenge of measuring the most important mechanical quantities in living subjects.
- •
Our current understanding of the role of mechanics in osteoarthritis is based largely on studies of deviations from normal mechanics caused by malalignment, injury, and deformity.
- •
Some treatments for osteoarthritis focus on correcting mechanics, but there appears to be scope for more mechanically based interventions for osteoarthritis.
Introduction
Mechanics play a role in the initiation, progression, and successful treatment of osteoarthritis (OA). However, not enough is known about which specific mechanical parameters are most important and what their impact is on the disease process, so comprehensive statements cannot be made about how mechanics should be modified to prevent, slow, or arrest the disease process. The idea of a mechanical role in OA is often made clear to the patient: OA is wear-and-tear arthritis, and joint surfaces need to be replaced because of wear in advanced stages of the disease, much like bearing surfaces in an engine that have worn down after too many revolutions. The parallels with machines are sometimes (at least superficially) obvious: obese people load their joints more and have a higher prevalence of OA, which is analogous to higher loads on a bearing increasing the rate of wear on its surfaces, a well-known mechanical phenomenon. Sufficiently high loads can destroy any tissue, so there is a level of joint loading that can injure cartilage irreversibly, leading to erosion from the joint surface. However, the usefulness of drawing parallels with machines is limited. Cartilage is avascular and aneural, but it is a living tissue with the capacity to adapt to its mechanical environment; it endures apparently unscathed through the most active decades of life in most people. Many surgical procedures and other treatment and prevention approaches are currently based implicitly or explicitly on the assumption that they improve or correct joint mechanics, and that this improvement or correction is required to protect the joint from OA. Justifying and improving mechanically based treatment and prevention approaches requires a critical understanding of the methods used to study joint mechanics and the current evidence for the role of mechanics in OA. This article summarizes methods for assessing joint mechanics and their relative merits and limitations, describes current evidence for the role of mechanics in OA initiation and progression, and describes some current treatment approaches that focus on modifying joint mechanics.
Introduction
Mechanics play a role in the initiation, progression, and successful treatment of osteoarthritis (OA). However, not enough is known about which specific mechanical parameters are most important and what their impact is on the disease process, so comprehensive statements cannot be made about how mechanics should be modified to prevent, slow, or arrest the disease process. The idea of a mechanical role in OA is often made clear to the patient: OA is wear-and-tear arthritis, and joint surfaces need to be replaced because of wear in advanced stages of the disease, much like bearing surfaces in an engine that have worn down after too many revolutions. The parallels with machines are sometimes (at least superficially) obvious: obese people load their joints more and have a higher prevalence of OA, which is analogous to higher loads on a bearing increasing the rate of wear on its surfaces, a well-known mechanical phenomenon. Sufficiently high loads can destroy any tissue, so there is a level of joint loading that can injure cartilage irreversibly, leading to erosion from the joint surface. However, the usefulness of drawing parallels with machines is limited. Cartilage is avascular and aneural, but it is a living tissue with the capacity to adapt to its mechanical environment; it endures apparently unscathed through the most active decades of life in most people. Many surgical procedures and other treatment and prevention approaches are currently based implicitly or explicitly on the assumption that they improve or correct joint mechanics, and that this improvement or correction is required to protect the joint from OA. Justifying and improving mechanically based treatment and prevention approaches requires a critical understanding of the methods used to study joint mechanics and the current evidence for the role of mechanics in OA. This article summarizes methods for assessing joint mechanics and their relative merits and limitations, describes current evidence for the role of mechanics in OA initiation and progression, and describes some current treatment approaches that focus on modifying joint mechanics.
Mechanical hypotheses about OA
Many of the hypotheses proposed to explain why OA begins and progresses center on mechanics. A widely accepted hypothesis regarding OA pathogenesis is that acute trauma destroys chondrocytes and disrupts the extracellular matrix, resulting in proteoglycan depletion, cartilage breakdown, and subsequent OA. Surface damage, proteoglycan loss, and chondrocyte death are seen commonly in OA, and similar observations have been made with in vitro impact studies on human and animal specimens. Recent research suggests that even moderate loading can induce biologic damage at levels less than those required to produce detectable macroscopic damage, and could be an early event in OA pathogenesis. Prolonged exposure to overloading caused by obesity and/or altered joint mechanics are also hypothesized to be detrimental to long-term joint health.
Altered kinematics and associated increased shear stress, in particular are thought to be key initiating factors explaining idiopathic forms of OA. In the articulating joint, cartilage is exposed to compressive loading (tending to compress the cartilage) and simultaneous shear loading (tending to change the shape of the cartilage) ( Fig. 1 ) as the 2 cartilage surfaces slide relative to one another. Abnormal motion (perhaps caused by an anterior cruciate ligament [ACL] injury, joint laxity, or aging) results in a shift in the region of loading to a cartilage zone not conditioned to frequent load bearing. This shift results in cartilage fibrillation at the cartilage surface, which is commonly seen in early stages of OA. Following fibrillation there is an increase in friction that increases the tangential shear load at the cartilage surface, resulting in increased shear stress in the collagen network and further fibrillation. Cartilage metabolic activity depends on the type of loading (eg, hydrostatic compression, shear) and exhibits a particularly negative response to excessive shear stress. Because of fibrillation, friction, and increased shear stresses, there is a metabolic upregulation of catabolic factors detrimental to cartilage health (eg, matrix metalloproteinase and interleukins), resulting in further cartilage degeneration.

Another group of hypotheses suggest that OA progresses because of mechanical changes in subchondral bone. An early view was that, because of impulse loading (loading that changes rapidly over time) and cumulative trauma, microfractures are created in the trabeculae that are repaired by fracture callus. As the trabeculae become thicker and new trabeculae are added, the repair process stiffens the subchondral bone, in effect acting as a support for the end plate, which causes the subchondral region to become less compliant, resulting in increased cartilage stresses and eventual degradation. Variable subchondral bone stiffening across the joint surface also results in stiffness gradients and subsequent increased cartilage shear stresses. A more recent, and generally accepted, hypothesis speculates that microfractures within the subchondral cortical end plate (as opposed to adjacent trabeculae), result in subchondral thickening. These microfractures, attributed to impulse loading and repetitive stress, are hypothesized to increase biological activity at the site of injury and result in increased bone turnover and reactivation of the secondary center of ossification, resulting in subchondral thickening, cartilage thinning, and increased cartilage stresses.
Although evidence is emerging from animal studies in support of some of these hypotheses, little work has been done in humans. Studies are emerging that examine the role of bone in OA using traditional bone mineral density (BMD) measures. For example, cartilage thinning correlates with bone structure losses in the contralateral compartment in subjects with tibiofemoral OA. Ratios of medial to lateral compartment BMD have also been associated with compartmental tibiofemoral OA. In particular, higher BMD in the medial compartment was associated with medial joint space narrowing, and medial sclerosis and higher BMD in the lateral compartment was associated with lateral joint space narrowing and lateral sclerosis. However, it is important to note that these findings were made using two-dimensional measurements of bone density, and similar findings were not found when using tomographic methods. Improved tools for measuring bone, cartilage, and joint mechanics may ultimately allow the testing of these and other hypotheses in vivo in humans.
Methods for assessing joint mechanics
The Challenges of Study Design
It is difficult to test hypotheses about the role of mechanics in OA initiation and progression and the effects of changes in mechanics on OA because appropriate in vivo human studies present major challenges. First, studies require a well-characterized population that has, or is at risk for, OA, and such populations can be difficult to identify. Large populations are generally needed for studies to have appropriate statistical power, which adds to the cost and difficulty of managing the study. Second, studies require a means of assessing OA incidence or progression. Radiographic measurements of OA (eg, Kellgren-Lawrence grade) are often insensitive to early OA and to small increments of progression. Advanced magnetic resonance imaging (MRI) techniques have shown potential for more sensitive, quantitative measurement of OA progression, but these measures are more time-consuming and expensive than radiographic approaches. Most critically, such studies require in vivo assessments of joint mechanics. Standard clinical measurements used as surrogates for mechanics have been employed in several studies, but they have many limitations. More direct measurements of mechanics (with fewer limitations than the clinical surrogate measures) have been developed, but studies have generally only been done in small populations because the measurements are expensive and time consuming.
What Do We Need to Measure and What are the Obstacles?
The hypotheses about the links between mechanics and OA, and these hypotheses define the mechanical quantities of primary interest to researchers. However, the most interesting quantities are among the most difficult to measure. Compressive and tangential shear forces on the joint surfaces are key quantities in many hypotheses, but they can only be measured directly by implanting a measurement device into the joint, which is too interventional (in the case of the natural joint) for most in vivo studies of humans. Force distribution on the cartilage surface is also widely thought to be important: a moderate force transmitted through a small contact area may produce local stresses that cause cartilage damage, whereas the same force transmitted through a large contact area would produce no cartilage injury. Although there are sensors available to measure force distribution, they must also be implanted, which carries the same limitations in vivo as direct measurements of force. Loading rate has been postulated to play a role in OA. Assessing loading rate requires rapid measurements of force (typically hundreds per second), which is more challenging than static force measurements. There is also interest in how force is transmitted through joint structures such as cartilage and bone (stress). Measuring stress in simple machines and structures is a challenge, and stress has not been measured in joints in vivo. Some approaches are emerging for measuring strain, or deformation of the joint tissues in response to stress, but the relationship between stress and strain is more complex in joint tissues than in, for example, steel or glass, which makes it difficult to use strain measurements to predict stress. Kinematics (joint movement) are easier to measure and several methods for accurately quantifying joint kinematics in vivo have been developed. Kinematics describe how the bones that make up the joint move relative to each other, which can reflect where load is transmitted through the surfaces and the lines of action of structures that transmit forces.
Ex Vivo Studies and Joint Models
Current understanding of joint mechanics is founded on ex vivo studies, which are inappropriate for linking mechanics with clinical symptoms. In ex vivo studies, kinematics and contact mechanics have been measured in cadaver specimens loaded in mechanical rigs. Although studies of this type have improved understand of the biomechanics of healthy joints, their central limitation for studying OA is that morphologic adaptations caused by the disease process or the healing process and mechanical links to clinical symptoms such as pain and ongoing processes such as cartilage degeneration cannot be studied in cadavers. An alternative that avoids some of the limitations of mechanical measurements that can be made in vivo is to predict joint mechanics using mathematical models. Models are limited primarily by the assumptions that must be made to formulate them. Models incorporating sophisticated descriptions of joint structures have been developed, validated, and used to answer specific clinically motivated questions. Two primary limitations of mathematical models are that (1) many simplifying assumptions must be made about the properties of the joint, which limits their validity and applicability; and (2) like ex vivo studies, they are inappropriate for studying links with ongoing processes and symptoms in vivo, unless these changes are measured and incorporated into the model.
Subject-specific finite element (FE) modeling is an emerging technique in musculoskeletal research that has potential for providing information on the role of mechanics in OA initiation and progression. FE modeling is a computational engineering technique used to evaluate how a structure with complex geometry and composed of a range of materials behaves when subjected to loading. Its basic premise is to divide a complicated object into a finite number of small, manageable pieces (elements). The behavior of each element can be described mathematically and evaluated computationally. Using clinical computed tomography (CT) or MRI, a joint’s bone, cartilage, and soft tissue geometry can be acquired. Tissue material properties (eg, elastic modulus, stiffness) can also be estimated using CT or MRI. Image-derived geometry and material properties can then be used to create a subject-specific FE model that can be analyzed under varying loading scenarios (eg, repetitive walking, impact-intensive running) to simulate bone and cartilage responses to loading. Unique information that is impossible to measure experimentally (eg, internal stress and strain distributions in both bone and cartilage) can be acquired using FE modeling and linked with in vivo symptoms and OA processes. The FE method can also be applied longitudinally to evaluate bone and cartilage structural behavior following OA-related morphologic and mechanical alterations to these tissues. However, application of subject-specific FE modeling to address OA-related questions is in its infancy. To date, research using subject-specific FE modeling has been primarily restricted to addressing osteoporosis-related research questions (eg, fracture strength) of bony structures not necessarily affected by OA (eg, radius, femoral neck). This lack of OA-related FE research is likely due to difficulties associated with validating FE models comprising numerous complex joint tissues. Validating models requires direct measurements of mechanical quantities such as stress and strain, which, as we have described earlier, are difficult to measure directly. However, new methods have recently been developed that have potential to validate internal strain distributions in bone and cartilage acquired using FE modeling, and that can be applied using intact joints. These developments, combined with improvements in CT and MRI technologies and computing power, have made subject-specific FE modeling a candidate technique for addressing specific questions regarding the role of mechanics in OA initiation and progression. However, application of the approach to large populations is limited by long data analysis (segmentation) and processing times and the challenge of model validation.
Radiographic Measures of Alignment
Most of the measures used clinically to quantify joint mechanics assess joint alignment. For example, tibiofemoral alignment is often quantified with the femorotibial angle, or hip-knee-ankle angle. A range of measures has emerged for quantifying patellofemoral alignment, with particular emphasis on medial-lateral position and patellar tilt. A central limitation of this approach is that the measures describe the joint (whose primary function is to move) at only 1 static position. In addition, although it is intuitive that these alignment measures are related to how load is transmitted in the joint, for most measures it is unclear by how much any given change in alignment measure would change force distribution in the joint. A further limitation is that the accuracy and repeatability of these measures are affected by their two-dimensional nature. Two-dimensional radiographic measurements are prone to errors caused by magnification and subject positioning. MRI and CT collect three-dimensional information about joint anatomy, which has been used in such applications as quantifying femoral neck deformities thought to be associated with hip OA. However, in many cases the three-dimensional data are still reduced to a two-dimensional measurement, which does not adequately describe three-dimensional deformities.
Gait Analysis
Gait analysis (often more generally referred to as motion analysis) is an important modality for estimating joint mechanics in activity. In motion analysis, movement of the joint segments is tracked (most often with an optoelectronic system), and loads applied to the body (eg, ground reaction forces) are measured. Mechanical analysis can then be used to assess the resultant forces and moments at the joints for each position for which a complete set of measurements is available. The resultant forces and moments at the joints, which are output by most commercial motion analysis systems, are different from the contact forces (also known as the bone-on-bone forces) in the joint. Determining contact forces requires further analysis using joint models and the measured joint segment positions and loads applied to the body. One advantage of gait analysis is that movement is unconstrained by the measurement system, and therefore a large range of activities can be studied. One key limitation of gait analysis is that joint segments are typically tracked with markers fixed to the skin that move substantially relative to the bones. However, it is often difficult to identify the joint center (or axis of rotation), particularly at the hip, and it is difficult to assess how well the measured marker positions correspond with motion at the joint (differences have been found to be on the order of 10° for knee flexion ). The use of large groups of skin-mounted markers has reduced the error caused by skin movement, although error will still be influenced by body habitus. A second key limitation is that the models and analysis needed to determine joint contact forces require many simplifications and assumptions, limiting the accuracy with which these loads can be measured. Models have often been based on the anatomy of a single specimen or participant, as opposed to using subject-specific anatomic measurements, which limits the usefulness of these models, particularly when pathologic joints are involved.
Roentgen Stereophotogrammetric Analysis and In Vivo Radiography
Some of the limitations of motion analysis have been addressed with radiography-based methods for measuring motion, including roentgen stereophotogrammetric analysis (RSA; also known as radiostereometric analysis) and fluoroscopy. Three-dimensional knee kinematics have been measured during activity in vivo using single-plane fluoroscopy and subsequent image processing. This measurement has been done in joints after arthroplasty and in natural joints. A limitation of the single-plane approach is that measurement errors out of the imaging plane are large. More accurate three-dimensional measurements of kinematics can be made with biplanar fluoroscopy and biplanar radiography, which have been used to study kinematics in the knees, hip, shoulder, and other joints. Although many biplanar radiography studies have been done using a series of static positions, high-speed biplanar radiography has made possible accurate measurements of kinematics during dynamic activity. Because these measurements are so accurate, combining kinematics with known joint geometry yields predictions of joint contact interactions. In some approaches, markers (typically small tantalum spheres) have been implanted into the bones, which is an invasive procedure and therefore a limitation. However, recent work using model-based approaches suggests that measurements can be made accurately and precisely enough to be clinically useful without using implanted markers. A key limitation of all these approaches is that they expose patients to ionizing radiation. Radiation doses vary with the joint of interest and the size of the subject. Such exposure always carries some risk and limits the number of repeat assessments that can be made, although, in general, imaging of the extremities is less of a concern than whole-body imaging because the body core can be shielded from radiation.
MRI Measurements of Kinematics
MRI has been used to assess joint kinematics and has the advantages that soft tissues are easily visualized and that no ionizing radiation is required, making it ideal for use in longitudinal studies. The different MRI-based approaches for assessing kinematics are distinguished from one another other by whether they describe two-dimensional or three-dimensional movement, whether they are assessed while the joint is actively loaded, and whether the movement is measured continuously. Many MRI studies of kinematics have focused on the patellofemoral joint, largely because patellar tracking is difficult to measure with other techniques such as gait analysis because of difficulties tracking skin-mounted markers on this small bone. Two-dimensional techniques have been used to assess kinematics at sequential static angles of knee flexion, dynamically over a range of knee flexion, and unloaded and loaded in a supine, lateral, or standing position. Loading in the supine or lateral position is performed by applying, respectively, an axial force to the foot or a torsional load to the shank using custom-designed rigs. The primary limitation of two-dimensional studies is that they neglect at least half of the movement, because describing any joint’s movement completely requires 6 quantities of movement (typically 3 rotations and 3 translations). Planar studies can measure a maximum of 3 quantities.
Three-dimensional MRI-based kinematic methods, which measure the 6 quantities of movement, have also been developed and can be categorized in the same way as the two-dimensional techniques (sequential static vs dynamic, unloaded vs loaded). The static three-dimensional methods have used standard MRI sequences, whereas the dynamic methods have used ultrafast gradient echo, cine-MRI, phase-contrast MRI, and spoiled gradient echoes with radial acquisitions. Although promising, these techniques have some limitations, most notably that static postures in a supine position do not necessarily represent normal activities of daily living, and dynamic methods either require subjects to extend their knees very slowly, which is not necessarily representative of normal motion, or to flex and extend their knees through many cycles that are averaged, which can cause errors if the motion is not repeated perfectly between cycles. Accuracy of these techniques ranges from approximately 1 mm and 1° to approximately 2 mm and 3°. Clinically important differences are likely in this range. Not all techniques have been rigorously validated and, therefore, care should be taken when interpreting data.
There are inherent trade-offs between static and dynamic methods and loaded and unloaded methods, as well as noteworthy limitations to these techniques. Substantial differences in two-dimensional patellar kinematic measures have been observed between unloaded, sequential static assessments and unloaded, dynamic assessments. Substantial differences were similarly observed between static, loaded assessments and dynamic, loaded assessments in a three-dimensional analysis. These results suggest that differences exist between static and dynamic cases, regardless of applied load. To further support this, 2 other studies have shown differences in two-dimensional patellar kinematics assessed dynamically between a supine low-load scenario and an upright 45% body weight scenario and in three-dimensional patellar kinematics assessed statically in the supine position at 0%, 15%, and 30% body weight scenario. Most methods apply low loads to the limb (0%–50% body weight load, open chain and closed chain), because of the limitations of loading the leg in the closed-bore MRI scanner. Open configuration MRI allows greater loads to be applied because the subject can stand upright (at least 45% body weight in weightbearing). However, these scanners have lower field strengths, limiting image quality and increasing scan times, and are not widely available. The range of flexion angles that can be studied is also limited by the MRI scanner configuration. Depending on knee size, flexion angles of up to approximately 50° can be assessed in closed-bore systems. With open configuration scanners, angles of 60° and greater can be assessed. In addition to these differences, the definition of the kinematic quantities differs between studies, which makes comparing results between studies challenging. An additional limitation is that most of this work has focused on the knee; few data are available for kinematics of the hip, shoulder, elbow, or hand.
MRI Measurements of Contact Area
Joint contact areas are fundamental to the understanding of load transmission through the joint and its relationship to local damage in OA. Contact areas, contact loads, contact stresses, and tissue stresses would ideally be measured. However, currently the only measure possible in vivo is contact area. Contact area measurements are useful because they provide information about where loads are transmitted on the cartilage surface and they can be used to find average contact stress (if joint loads can be estimated). Several groups have developed methods of assessing contact area in vivo from MRI. All of these methods have used gradient echo techniques with fat suppression, which is optimized to view cartilage. These scans have been acquired using traditional scanners (1.5 and 3.0 T) and open-configuration scanners (0.5 T) using the loading methods similar to those used for kinematic assessments. Contact area is generally assessed at sequential static, loaded angles of knee flexion, although it was measured during continuous flexion in a recent study. Axial and sagittal scans have been used. The chosen plane should be the one that provides the most information about the contact periphery. Once the MRI scans are acquired, they must be processed to calculate contact areas, which can be done by delineating the contact region in a slice-by-slice manner and multiplying the length of each contact line by slice thickness or interpolating between slices and summing the areas of discrete patches. Alternatively, adjacent cartilage plates can be segmented separately and contact can then be calculated by either expanding 1 surface by a pixel and defining overlap as contact or by performing a proximity analysis. The coordinates of the contact centroid have also been reported on occasion. These may provide valuable information about changes in contact location and are most useful when reported in a relevant coordinate system, such as that used for kinematic assessment. Validation of MRI-based techniques has been limited. Only 3 studies have examined agreement with a reference standard, 2 using cadaver specimens, and 1 using a phantom. Errors, expressed as coefficient of variation, were found to range from 3% to 11%. Intraobserver, interobserver, and intrasubject repeatability have also been assessed in vivo and have ranged from 3% to 10%. Many of the factors that affect measures of patellar kinematics, such as loading, range of motion, and tibiofemoral angle, are equally applicable to measures of contact area. Most studies have focused on the patellofemoral joint, whereas others have examined contact areas in the elbow and tibiofemoral joint. Little or no work has addressed the hip, shoulder, wrist, or hands.
The Future
Technologic advances hold promise for more and better in vivo mechanical measurements. Many of the methods described in this article are now beginning to be used to study patient populations. One area with promise is MRI mapping of cartilage strain, which had been done ex vivo in the knee and the hip. This work is challenging because achieving the resolution required to detect small changes in cartilage thickness in a short imaging time requires scanners with very high field strengths. As these scanners become more widely available for clinical use, there will be the potential to begin assessments of strain in vivo. Groups are also beginning to integrate mechanical measures with other OA disease indicators. For example, a recent study combined measures of contact area with measures of T1rho and T2 relaxometry; individuals with OA had longer T1rho and T2 relaxation times and greater contact areas. Integration of mechanical measurements with MR techniques designed to detect early degenerative changes may be useful for improving understanding of the causes of OA. CT technology is also improving, with scans becoming faster and requiring lower doses of ionizing radiation. This progress raises the possibility of wider use of CT to assess joint kinematics and other mechanical quantities as well as integrating these measures with radiographic and MRI-based measures of tissue degeneration and OA. In addition, developments in nanotechnology suggest the feasibility of developing implantable transducers that transmit measurements through telemetry. This has been done on a limited scale but, as technology improves, it may become more widespread.
Current evidence for the role of mechanics in OA initiation and progression
Distinction Between Normal Aging and OA
OA is often thought of as a disease of aging that is mechanical in origin. However, it is likely that there are some changes in mechanics over time that are a result of normal aging and not necessarily the OA disease process. For example, 1 study found, in participants without radiographic signs of OA, a significant increase in valgus alignment with age at a rate of 0.03° and 0.04° per year in men and women, respectively. In the same study, participants with OA tended to show increasing varus angulation. Another study showed, through principal component analysis, that patterns of gait differed between young (23.9 ± 2.6 years) and older (65.5 ± 5.2 years) participants when climbing stairs. These findings suggest that patterns of changes in joint mechanics may differ not only with OA but also with normal aging, which highlights the need for establishing age-dependent population norms for studying OA.
Alignment
Joint malalignment or incongruence leads to altered loading patterns at the cartilage surface, and joint alignment is therefore associated with OA. Tibiofemoral alignment has been studied extensively in individuals who have tibiofemoral and/or patellofemoral OA. However, this relationship is not necessarily straightforward, with 1 study reporting that tibiofemoral alignment did not predict incident tibiofemoral OA, suggesting that instead tibiofemoral alignment may be a marker of disease or progression. Another paradigm is that increased body mass index is a risk factor for incident OA but in one study it only affected OA progression in knees with moderate malalignment (odds ratio [OR] 1.23), not neutral knees. A subsequent study showed that a high body mass index increased risk of OA progression only in neutral and valgus knees (OR 1.4 and 1.8, respectively). These results are conflicting, indicating that further examination is necessary. Regardless, tibiofemoral alignment has also been shown to be protective against cartilage loss in the less loaded compartment, as assessed by MRI (OR 3.7 and 6.0 for the protected lateral and medial compartments, respectively). Tibiofemoral alignment has also been shown to be associated with patellofemoral OA. Varus tibiofemoral malalignment increases the odds of medial compartment radiographic patellofemoral OA progression (OR 1.85) and valgus tibiofemoral malalignment increases the odds of lateral compartment patellofemoral OA progression (OR 1.64). More recently, the relationship between alignment and cartilage tissue properties, as assessed noninvasively by MRI, has also been studied. For example, in a study using delayed gadolinium-enhanced magnetic resonance imaging of cartilage as a measure of cartilage health, knees in valgus tended to have lower dGEMRIC values (less proteoglycan) in the lateral compartment, and knees in varus tended to have lower dGEMRIC values in the medial compartment. T2 relaxation times have also been shown to be increased in the medial compartment of individuals with varus malalignment. Together, these data suggest that tibiofemoral alignment plays an important role in OA but, in some instances, this role is part of a complex interaction with other mechanical risk factors.
Patellar alignment, along with tibiofemoral alignment, plays an important role in patellofemoral OA. Lateral patellar alignment has been associated with lateral joint space narrowing (OR 8.26), lateral osteophytes (OR 3.07), lateral cartilage loss (OR 3.4), and lateral bone marrow lesions (OR 3.2). Medial patellar alignment has been associated with medial joint space narrowing (OR 2.85). In a study of OA progression, medial displacement of the patella increased the risk of medial patellofemoral joint space narrowing progression (OR 2.2), whereas it was protective of lateral patellofemoral joint space narrowing progression (OR 0.4), and increasing lateral tilt was protective of medial patellofemoral joint space narrowing progression (OR 0.2). It has also been shown that patella alta (a high-riding patella) is associated with lateral joint space narrowing (OR 2.77), lateral osteophytes (OR 1.67), medial and lateral cartilage loss (OR 2.0–2.4 and 1.5–2.0, respectively), and medial and lateral bone marrow lesions (OR 2.5–2.9) and medial and lateral subchondral bone attrition (OR 2.2 and 3.5, respectively). There is a high prevalence of medial patellofemoral OA that is independent of alignment. This highlights that alignment alone does not explain all patellofemoral OA and perhaps more complex techniques, such as assessments of three-dimensional kinematics and contact area, may be required to understand the relationship between mechanics and patellofemoral OA.
Other Surrogates for High Joint Forces
Several studies have found associations between surrogate measures of high joint forces and OA. As discussed previously, joint forces cannot be measured directly, but estimates based on anthropometry, joint congruency or alignment, gait analysis, and activities of daily living can be used to study the effect of higher joint forces. Several mechanical factors have been associated with radiographic and/or MRI-based definitions of OA such as obesity, patellar alignment, knee height, squatting, and meniscectomy. Although these are not direct measures of mechanics, these associations suggest that mechanics play a role in OA. For example, knee height, which contributes to increased moments about the knee and associated higher theoretic contact forces in the joints, was associated with an increasing prevalence of symptomatic and radiographic knee OA. More specifically, increased knee height, measured from the ground to the femoral condyles when sitting, was associated with radiographic patellofemoral OA in men (OR 1.7) and was associated with symptomatic patellofemoral OA in women (OR 2.2). Prolonged squatting (which theoretically produces high forces in the knee) in elderly Chinese men was also associated with higher risk for knee OA. Obesity has been identified as a risk factor of both patellofemoral OA and tibiofemoral OA. Obesity puts individuals at greater risk of radiographic patellofemoral OA than radiographic tibiofemoral OA (OR 3.5, 7, and 1.9 for isolated patellofemoral, combined, and tibiofemoral OA, respectively). Gait analysis is probably the most widely used tool to estimate joint loads in OA. One of the strongest relationships established using gait analysis is that knee adduction moment is associated with OA. A more recent study showed that cumulative knee adductor load was better than the peak knee adduction moment at discriminating between healthy and OA groups. Gait analysis has also revealed that mechanics at other joints such as the hip and ankle can affect OA at the knee. For example, medial tibiofemoral compartment OA is associated with hip adduction moments and higher axial loading rates at the hip, knee, and ankle. Gait parameters at the hip, knee, and ankle also vary, depending on the severity of OA. It has been suggested that the changes in gait mechanics may be a compensatory mechanism to reduce pain by reducing loads at the affected joint. Asymmetric gait patterns have also been shown in subjects who have advanced hip OA and in subjects with unilateral knee OA. In the latter study, individuals with bilateral OA did not display the same asymmetry in gait patterns. Ankle mechanics have been studied to a lesser extent than knee and hip mechanics, but it has been shown that a greater toe-out angle and load is associated with the progression of medial tibiofemoral OA. All these surrogate measures of joint loading provide information about the relative magnitudes of force being transmitted through the joint, but they do not indicate where on the joint surface these loads are transmitted. Advances in weight-bearing imaging may provide this information in the future.
Injury
Joint fractures and acute injury to the menisci and ACL are all associated with the development of OA, but the specifics of how injury affects mechanics, and how mechanical changes lead to OA, are not clear. Fractures of the joint surface at the hip, knee, and ankle are associated with high rates of OA, and the development of OA is related to increased contact stress caused by the fracture. Meniscal and ACL injuries are well-known factors in the development of early knee OA. Forty-three percent of subjects who had undergone meniscectomy because of meniscal tears had radiographic evidence of OA after 16 years, meniscectomy increases risk of developing OA (OR 2.6), 51% of female soccer players with ACL injury had radiographic evidence of OA after 12 years, and 41% of male soccer players with ACL injury had radiographic evidence of OA after 14 years. A study of ACL injury in a population of individuals with symptomatic knee OA found that a complete ACL tear increased the risk for medial tibiofemoral cartilage thinning compared with those with intact ACL or partial ACL tears (OR 1.8, adjusted for age, body mass index, and gender). However, once an adjustment for medial meniscal tears was included in the regression model, a complete ACL tear was not an independent risk factor of cartilage thinning (OR 1.1). Bone marrow lesions were associated with ACL tears in the same cohort. Although these acute injuries are associated with OA, and change in joint mechanics produced by the injuries plays a role in the causes of OA, many other factors related to the patient, injury, and treatment affect whether OA develops after injury, and at what rate. Researchers have proposed hypotheses detailing the cascade of events involving the effect of ACL injury on knee mechanics, the resulting change in loading patterns, and the cartilage response.
Deformity
In contrast with knee OA, hip OA is generally associated with either instability or deformity, with primary hip OA considered rare. Although the association between severe hip deformity and OA has been understood for decades, interest has more recently focused on the role of more subtle deformities. It is well accepted that many cases of idiopathic hip OA can be attributed to bony deformity. The hypothesis that femoroacetabular impingement (FAI – contact between the femur and acetabulum due to deformity and/or extreme movement) is a major causal factor leading to hip OA has growing support in the orthopedic literature. Two mechanisms by which the cartilage and labrum are affected by FAI have been described. Cam impingement is a result of a nonspherical femoral head abutting against the acetabular rim in flexion and internal rotation ( Fig. 2 ). The abutment causes damage to the anterosuperior acetabular cartilage. Pincer impingement occurs as a result of linear contact between the femoral head-neck junction and the acetabular rim. In support of the FAI hypothesis, many studies have shown associations between features of impingement and cartilage damage. For example, in a study of 149 hips with mild or no radiographic OA, patients with radiological features of cam impingement (26 hips) had damage to the anterosuperior acetabular cartilage, whereas patients with radiological features of pincer impingement (16 hips) had a narrow strip of circumferential cartilage damage. Specific radiological features characteristic of FAI predicted progression to hip OA (over at least 10 years) in patients with a pistol-grip deformity. Early cartilage changes were present in hip cartilage in participants with cam deformities who had not complained of osteoarthritic symptoms. FAI is of particular concern given its high prevalence. The prevalence of cam deformities in young men was reported to be 24%, and the prevalence of specific cam and pincer deformity signs was higher in men than in women. Although hip deformity seems to be related to OA, it is not clear what dictates whether or not FAI deformities will lead to OA, how many patients with the symptoms of FAI have cartilage changes, at what stage osteoarthritic changes begin in FAI, how they progress, and whether they can be prevented or reversed.
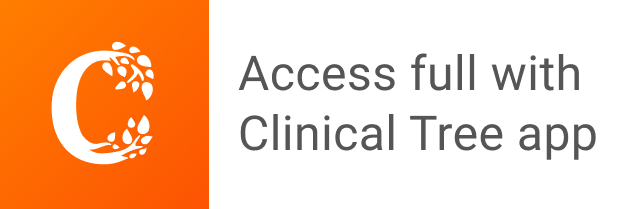