The Intervertebral Disk
Jeffrey C. Lotz, PhD
Aaron J. Fields, PhD
Andrew K. Chan, MD
Alexander M. Ballatori, BS
Dr. Lotz or an immediate family member has received royalties from Relievant MedSystems; has stock or stock options held in Bioniks, LLC, ISTO Technologies, Nocimed, LLC, Relievant MedSystems, and SMC Biotech; and has received research or institutional support from DePuy, A Johnson & Johnson Company, and Orthofix, Inc. Dr. Chan has received nonincome support (such as equipment or services), commercially derived honoraria, or other non-research-related funding (such as paid travel) from Orthofix, Inc. Dr. Ballatori or an immediate family member serves as a board member, owner, officer, or committee member of the American Academy of Orthopaedic Surgeons. Neither Dr. Fields nor any immediate family member has received anything of value from or has stock or stock options held in a commercial company or institution related directly or indirectly to the subject of this chapter.
Keywords: degenerative disk disease; disk degeneration; intervertebral disk; IVD; pathophysiology of intervertebral disk degeneration
INTRODUCTION
The intervertebral disk (IVD) is a fibrocartilaginous joint in the vertebral column that serves unique functional roles. It allows for multiaxial movement, while supporting the compressive forces exerted onto the spine. IVD degeneration is age-associated and can be accelerated by multiple, integrated mechanical and biological mechanisms. IVD degeneration is thought to be a leading cause of back pain, either directly as a pain generator or indirectly via diminished biomechanical properties and consequent alterations in adjacent tissue stresses. The goal of this chapter is to provide a summary of the fundamental characteristics of the IVD and processes involved with physiologic (age-related) and pathologic (painful) degeneration.
ANATOMY
The spinal column is composed of 33 vertebrae comprising five distinct regions: the cervical, thoracic, lumbar, sacral vertebrae, and the coccyx. At the most cephalad extent, the cervical spine is composed of seven vertebrae and assumes a lordotic curvature. Below that, the thoracic spine is composed of 12 vertebrae and assumes a kyphotic curvature. Caudally, the lumbar spine is composed of five vertebrae and assumes a lordotic curvature. Together, these regions give rise to the “S”-shaped characteristic curve of the spinal column. Next, the five, fused sacral vertebrae form a portion of the pelvis. At the most caudal extent of the spinal column, four small, fused vertebrae form the coccyx. Each vertebra is composed of an anterior portion (vertebral body) and a posterior arch which is formed by the pedicle, facet, lamina, and spinous process (Figure 1, A). The vertebral body is composed of an inner region of cancellous bone surrounded by a thin shell of cortical bone.
Each successive vertebra is connected via multiple points: anteriorly via the IVD (separating the vertebral bodies) (Figure 1, B) and posteriorly via the facet joints. Additional soft-tissue structures provide passive support, including the anterior longitudinal ligament, the posterior longitudinal ligament, the ligamentum flavum, the facet joint capsule, the interspinous ligament, and the supraspinous ligaments (Figure 1, C). The spinal column is also stabilized by a set of paraspinal muscles that include the erector spinae that runs longitudinally on the dorsal surface of the spinal column and functions to extend the spine; the psoas that runs longitudinally on the ventrolateral surface of the spinal column and serves to flex the hip (bilateral contraction) or laterally bend the trunk (unilateral contraction); and the multifidus that connects intersegmentally to stabilize the spine by acting like a bowstring to maintain lordosis.
Except between the atlas (C1) and the axis (C2), the IVD separates each successive vertebral body and provides a unique combination of compressive stiffness and flexibility to support normal spine biomechanics. It is composed of an inner nucleus pulposus (NP) and an outer ring termed the anulus fibrosus (AF) (Figure 1, B). The NP serves as an osmotic pump to attract water and generate hydraulic pressure when subjected to significant loads during activities of daily living. The AF encapsulates the gelatinous NP and provides the mechanical support to both contain NP pressure and constrain intervertebral rotations. The outer AF is integrated with the vertebral rim via a fibrocartilage enthesis that consists of a thin layer of calcified cartilage, or “tidemark.” The end plate (EP) is a bilayer of cartilage and bone that separates the disk from adjacent vertebrae.1 The cartilage EP integrates with the inner AF to fully encapsulate the NP. The EP facilitates important but potentially conflicting biophysical functions: it must be strong and thick to resist significant loads but must also be permeable to favor chemical transport and disk cellular vitality.1
COMPOSITION
The disk extracellular matrix (ECM) is maintained by a mixed population of cells and is finely tuned to meet disk biomechanical demands. Although the basic biochemical components of the NP, the AF, and the EP are generally the same, that is, water, proteoglycans (PG), and collagens, their relative proportions differ between structural domains (Table 1),2 although these proportions change dramatically with aging and disk degeneration. The composition of the healthy ECM varies gradually along the radial direction with the central NP having the highest water/PG and lowest collagen/elastin content and the outer AF having the lowest water/PG and highest collagen/elastin content. Moving cranially and caudally, the EP has the lowest water/PG and the highest collagen content.
TABLE 1 Approximate Relative Proportion of the Four Main Extracellular Matrix (ECM) Components of the Adult Human IVD. | ||||||||||||||||||||||||
---|---|---|---|---|---|---|---|---|---|---|---|---|---|---|---|---|---|---|---|---|---|---|---|---|
|
The major PG of the IVD is aggrecan, a large molecule with a molecular weight ranging from 3,000 to 7,000 kDa. Aggrecan structure is comprised of a linear core protein with large numbers of sulfated glycosaminoglycan (GAG) side chains, which carry a net negative charge. These GAGs bind mobile ions (mostly sodium) and thereby generate an osmotic pressure that attracts water and produces physical pressure to support spinal compression. The tendency of the PG network to swell and generate osmotic pressure is resisted circumferentially by tension in the network of collagen fibrils in the AF, which holds the NP together and anchors the periphery of the disk to the subchondral bone. The fraction of aggrecans decreases with degeneration, as proteolytic degradation of the link protein by matrix metalloproteinases (MMPs) increases PG fragmentation.
Collagen type I and II make up approximately 90% of total IVD collagen.3 Each molecule consists of three polypeptide chains, or alpha chains, twisted into a triple helix (300 nm long, 1.5 nm diameter). Individual molecules assemble end-on-end and side-by-side to form microfibrils. The NP, EP, and inner AF consist almost exclusively of type II fibrils, which are very fine (10 to 100 nm in diameter) in comparison to the larger type I fibrils (100 to 200 nm in diameter) that predominate in the AF and increase in relative proportion peripherally. The differences in the relative proportions of type I and type II collagen among the NP, AF, and EP hint at the predominant loading experienced by each structure of the disk: collagen type I is usually found in tensile structures such as ligaments, whereas collagen type II is normal in compressed tissues like articular cartilage. In the NP, the collagen fibers are irregularly oriented and outnumber PGs by approximately 20:1; in the AF, they are organized into 10 to 25 unidirectionally aligned concentric lamellae, which encircle the NP and attach to the cartilage EP in the inner zone and to the vertebral EP in the outer zone (Figure 1, B). Alignment alternates between +30° and −30° with respect to the circumferential direction of the IVD.
The cartilage EP is typically between 0.1 and 2.0 mm thick4; however, its thickness is known to vary with position and level, being thinner centrally and in the upper levels of the spine than peripherally and in the lower levels of the spine.4 The nature of the structural integration between the EP and the surrounding tissues also varies with position. Peripherally, the collagen fibers in the lamellae of the AF are continuous with the collagen fibers in the EP, whereas centrally, the integration between collagen fibers in the NP and the EP is more convoluted.4 The collagen fibers of the cartilaginous and bony components of the EP are completely separate.4
Elastin is the third and relatively less abundant structural protein in the disk. These fine, extensible fibers predominate in the AF, where they are organized in a crisscross pattern between neighboring lamellae (Figure 2). This pattern suggests that elastin helps resist radial expansion of the NP during disk compression by regulating interlamellar sliding or shearing movements.
Advanced glycation end-products (AGEs), often called nonenzymatic “cross-links,” play structural and biological roles with increasing importance in the aging disk. One pathway of cross-link formation is via nonenzymatic glycation of
the free amino groups of proteins and lipids by reducing sugars. The cross-link formation is irreversible, and thus, long-lived proteins like collagen are especially susceptible to AGE accumulation. In normal disks, AGE accumulation has been shown to increase with age, trigger catabolic actions within disk cells, reduce disk hydration, and increase disk tissue stiffness.5 Hyperglycemic conditions like diabetes accelerate the ordinarily slow rate of endogenous glycation by increasing the availability of sugars that can attach to the amino groups leading to accelerated disk degeneration.5
the free amino groups of proteins and lipids by reducing sugars. The cross-link formation is irreversible, and thus, long-lived proteins like collagen are especially susceptible to AGE accumulation. In normal disks, AGE accumulation has been shown to increase with age, trigger catabolic actions within disk cells, reduce disk hydration, and increase disk tissue stiffness.5 Hyperglycemic conditions like diabetes accelerate the ordinarily slow rate of endogenous glycation by increasing the availability of sugars that can attach to the amino groups leading to accelerated disk degeneration.5
The most abundant nonstructural proteins in the IVD are the families of MMPs (matrix-metalloproteinases), TIMPs (tissue inhibitors of metalloproteinases), and ADAMTS (A Disintegrin and Metalloproteinase with Thrombospondin Motifs). These zinc-dependent proteinases, which are regulated in part by cytokines, increase during disk degeneration, leading to increased cleavage of the ECM.
Degenerative processes compromise both the biological and mechanical functions of the ECM. Enzymatic degradation of NP PG reduces osmotic pressure. This is typically concurrent with increased collagen production and increased AGE levels that combine to decrease swelling and water content. These ECM changes associate with age and can be accelerated by excessive physical loading,6 where adverse tissue stress can damage matrix and trigger altered biological activities, that in turn cause NP fibrosis and disorganization of the AF architecture. ECM biochemical changes and damage accumulation can stimulate inflammatory cellular responses that promote a cascade of further structural modifications that progressively compromise disk biomechanics.
BIOLOGY
Compared with other tissues, relatively few disk cells maintain an extensive ECM. IVD cells are a mixed population across the NP, AF and EP.7 At birth, the NP contains notochordal cells that are remnants of spinal development. In humans, notochordal cells are gradually replaced by chondrocyte-like cells over the first two decades of life. This conversion is initiated by notochordal cell apoptosis caused by a combination of increased pressure from gravity loading, plus decreases in nutrition secondary to changes in adjacent vertebral perfusion.8 Subsequently, cells from either the cartilage EP or inner anulus migrate to fill the NP.9
NP cells are chondrocytic and synthesize aggrecan and collagen II as the main structural proteins. By contrast, AF cells are fibrocytic and make type I, II, III, and VI collagen along with chondroitin and keratin sulfate. The cartilage EP is mainly composed of chondrocyte-like cells that mainly secrete PG and type II collagen.
The IVD is the largest avascular structure in the human body, with a distance of up to 6 to 8 mm between cells and the nearest blood supply.10 Therefore, nutrient supply and drainage of metabolic products rely on diffusion between the IVD cells and the capillary endings of the vascular network that terminates in the outer AF and in the bony EP. The diffusion rate depends on the microstructure and composition of the transport route, the rate of cellular consumption, and on the biochemical properties of the solute (size, shape, and charge). The EP has a higher permeability in the central NP zone, which is essential to maintain a sustainable metabolite transport to and from the NP cells. Competition for nutrients limits cell density to approximately 1,600 cells/mm.3 Because the disk tissue oxygen levels are low (0.5% to 5%), disk cells create energy via glycolysis, which utilizes glucose and generates lactic acid. Disk dehydration and EP sclerosis impair diffusion, as these changes reduce the effective pore size of the ECM and hinder solute transport.11,12 Impaired diffusion promotes cell death and disk degeneration because of nutrient deprivation, mainly oxygen and glucose, and accumulation of metabolic products, mainly lactic acid.
In addition to nutrient availability and the accumulation of metabolic waste products, disk cell function is influenced by spinal load and consequent matrix deformations. The healthy disk nucleus is largely water and, consequently, is subjected to hydrostatic pressure during spinal loading. NP cells can rapidly detect and respond to fluctuations in hydrostatic pressure. By contrast, the outer AF in the healthy disk is generally subjected to biaxial loading and AF cells are exquisitely sensitive to stretch. Matrix loading also stimulates cells indirectly by compacting matrix, which decreases tissue permeability, water content, oxygen tension, and pH. These changes can alter both PG and collagen synthesis. Data from both organ- and cell-level studies suggest the existence of optimal loading regimens, where both excessive and negligible pressure/stretch responses are catabolic, which may underlie the U-shaped relationship between physical exposure and back pain, where both low and high exposures are observed to be detrimental.
BIOMECHANICS
The spinal column supports the torso mass and facilitates trunk movement. By itself, the spinal column is inherently unstable, and it will buckle under axial compressive loading. In life, the column’s static and dynamic stability is determined by synergy between passive constraints (defined by osteoligamentous tissues such as the vertebra, facet joints, IVD, and intervertebral ligaments) and active constraints (defined by muscles such as the psoas, erector spinae, and multifidus). The passive constraints transmit the forces generated by muscle contraction. Additionally, the passive constraints provide for muscular antagonism, which enables the force of muscle contraction to re-elongate their antagonists. The contribution of passive and active stiffness varies at each spinal level.13 The clinical importance of active stiffness is demonstrated by the fact that the cross-sectional area of the paraspinal muscles is smaller in low back pain patients compared with healthy subjects.14 This may be because muscle weakness and fatigue leads
to changes in muscle recruitment patterns, increases in spinal force, and decreases in spinal stability.
to changes in muscle recruitment patterns, increases in spinal force, and decreases in spinal stability.
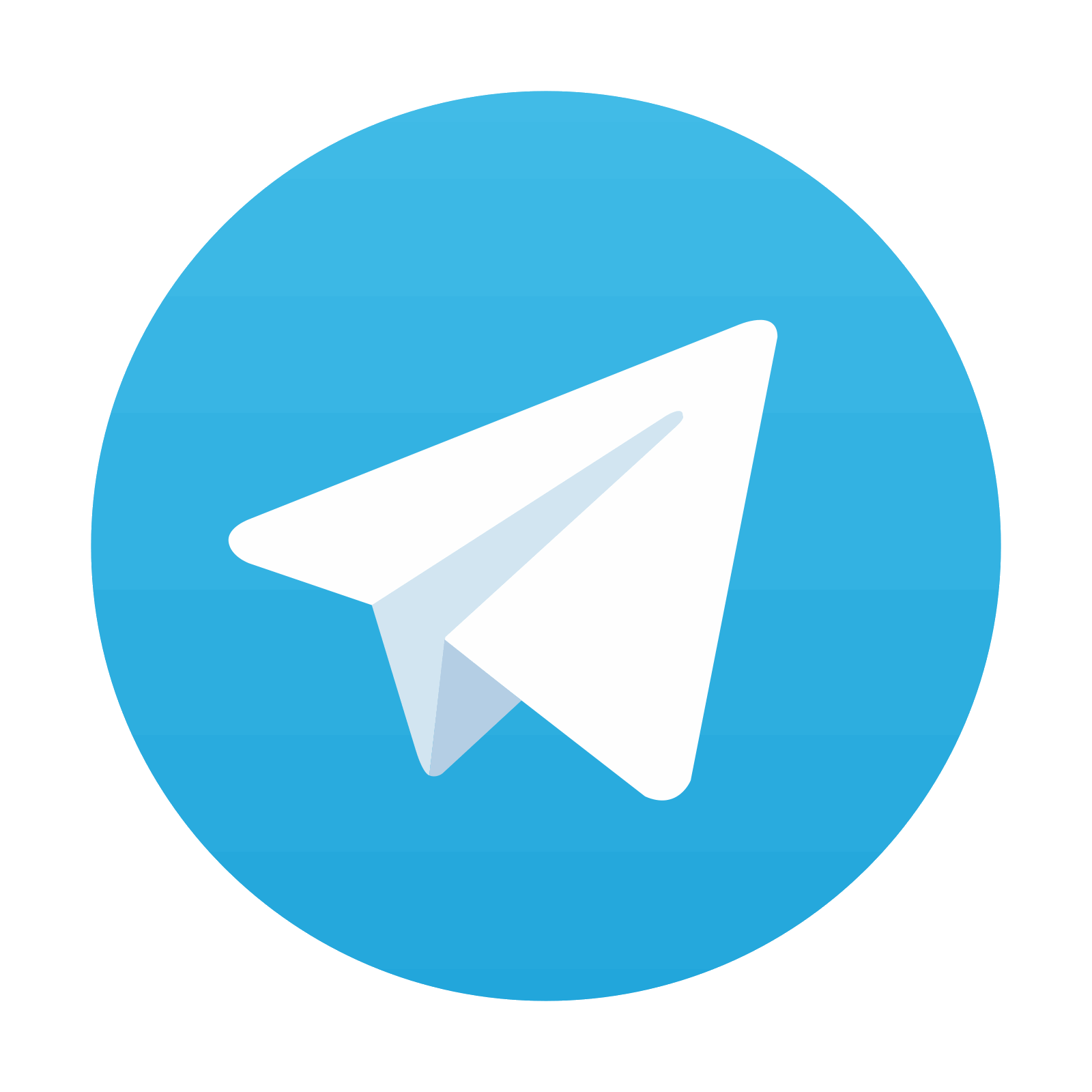
Stay updated, free articles. Join our Telegram channel
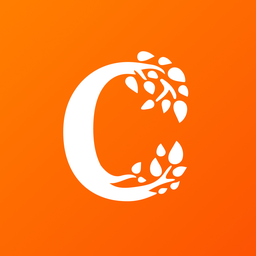
Full access? Get Clinical Tree
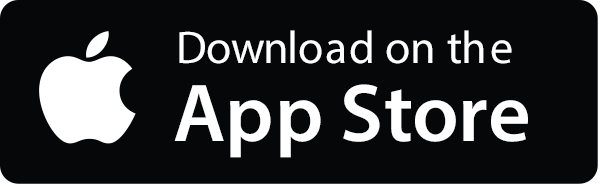
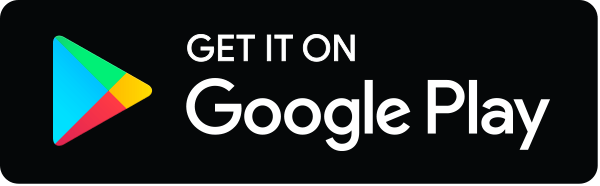