Examples of molecules previously identified in the Dupuytren Disease extracellular matrix
Structural and nonstructural ECM molecules
Matricellular (nonstructural) ECM molecules
Growth and differentiation factors
Collagens (I, III, + many others)
Decorin
Vitronectin
Hyaluronan (hyaluronic acid)
Proteases (MMP/ADAM/ADAMTS)
Protease inhibitors (TIMPs)
Laminin
Elastin
Fibronectin (including EDA)
Periostin
Tenascin C
CCN2 (CTGF)
CCN4 (WISP1)
Transforming growth factor ß (TGFß)
Tumor necrosis factor α (TNFα)
Basic fibroblast growth factor (bFGF)
Platelet-derived growth factor (PDGF)
Insulin-like growth factor-II (IGF-II)
6.4 Cellular Connections with the Extracellular Matrix
Connective tissue fibroblasts in general, and myofibroblasts in particular, obtain feedback from the biochemical and biomechanical components of their ECM (Hinz 2006; Tomasek et al. 2002) through specialized attachment points known as focal adhesions. While several different types of attachments have been characterized in in vitro settings, including focal complexes, fibrillar adhesions, and 3D matrix adhesions (Berrier and Yamada 2007; Harunaga and Yamada 2011), the primary cellular attachments that are formed in vivo are focal adhesions (Lock et al. 2008; Christopher and Guan 2000; Hinz et al. 2003) (Fig. 6.1). Focal adhesions are dynamically regulated multi-protein complexes of integrins and other proteins that span the cell membrane and connect cells to proteins in the Dupuytren Disease ECM, which include collagens (Magro et al. 1997a), laminin (Wilbrand et al. 2003), fibronectin (Magro et al. 1995), and matricellular molecules (Vi et al. 2009a). Both biochemical and biomechanical stimuli can activate focal adhesions to facilitate cellular responses. Many of these responses involve the polymerization of globular (G) actin monomers into the filamentous actins (F actin). Actin filaments bind vinculin and other focal adhesion proteins (Hinz and Gabbiani 2003a) to complete the structural link between the ECM and the cytoskeleton and facilitate ECM-induced changes in cellular motility, contractility, and substrate adhesion (Kawaguchi et al. 2003; Yamashiro et al. 1998; Hinz and Gabbiani 2003a).


Fig. 6.1
Three-dimensional confocal microscopy of Dupuytren Disease myofibroblasts cultured in type 1 collagen lattices. Stress fibers are stained with green Alexa 488 phalloidin, focal adhesions (vinculin immunoreactivity) are shown in red, and cell nuclei (DAPI staining) are shown in blue. The three-dimensional collagen matrix was visualized by laser reflectance microscopy, a technique that utilizes the reflection of laser light by the surfaces of collagen fibers (white). Changes in fluorescence intensity within and around these cells indicate cellular processes that are in or out of frame in this two-dimensional rendering of a three-dimensional image. These images illustrate the connections between matrix-associated collagen fibers, focal adhesions, and contractile stress fibers in myofibroblasts. Cells can be envisaged as prestressed lattice structures that are stabilized by the combination of tension and compression in accordance with the tensegrity principle (Ingber et al. 2014). changes in stress fiber length within cells are translated through focal adhesions to impose changes in collagen fiber density
6.5 Cellular Tensegrity Within the Extracellular Matrix
Myofibroblasts can be distinguished from other fibroblasts by their expression of a distinct form of filamentous actin known as α smooth muscle actin (αSMA) (Darby et al. 1990). When coupled to myosin in “stress” fibers, αSMA allows myofibroblasts to impose much greater contractile forces on the ECM through focal adhesions than connective tissue fibroblasts are normally capable of achieving (Hinz 2006). Myofibroblasts can also form intercellular (cell to cell) connections with other myofibroblasts through adherens junctions, further enhancing their capacity to coordinate ECM contraction in areas of high cell density (Follonier et al. 2008; Hinz and Gabbiani 2003b).
One way of visualizing cellular interactions with their surrounding matrix is to perceive cells as prestressed lattice structures that are stabilized by the combination of tension and compression. This concept, known as the tensegrity principle (Ingber et al. 2014), envisages actin/myosin stress fibers, intermediate filaments, and microtubules (α and β tubulin polymers) as tensional and compression elements that have analogous roles to the cables and columns that maintain the shape of a flexible structure. While a detailed description of cellular tensegrity is beyond the scope of this section (for details, see (Ingber et al. 2014)), it is nonetheless helpful for envisaging how external forces that pull on structural molecules in the ECM can cause integrins in focal adhesions linked to αSMA in stress fibers to distort the shape of a cell and stimulate cellular responses. This concept also works in reverse, allowing cells to contract the surrounding ECM by shortening the actin/myosin stress fibers in their cytoskeleton that are linked to integrins in focal adhesions and to structural molecules, such as collagens, in the ECM.
6.6 Extracellular Matrix Interactions and In Vitro Analyses of Dupuytren Disease Cells
Any molecule that promotes the coordinated and excessive contracture of the palmar fascia by myofibroblasts has potential as a therapeutic target. While many of these molecules may reside within palmar fascia fibroblasts or myofibroblasts, others elicit their signals through matricellular and other molecules to induce their effects through focal adhesions to modify cellular proliferation, myofibroblast formation, ECM secretion, or other disease-associated changes. For this reason, in vitro studies are performed on palmar fascia fibroblasts or fibrogenic myofibroblasts that do not include a physiologically relevant ECM risk, omitting the contributions of these interactions and, at worst, providing misleading information about cellular responses to treatments.
In practice, culturing cells in a physiologically relevant ECM is technically challenging, especially when most of the constituents of that ECM are yet to be characterized, as is the case in Dupuytren Disease. One approach to partially overcoming this hurdle is to collect the secretions of primary palmar fascia myofibroblasts and use them to “condition” collagen, hydrogel, or other relatively porous substrates in which cells can be cultured in three dimensions. While this approach can only provide an approximation of an ECM that is continually modified in vivo, nonetheless it has advantages over standard tissue culture plastic (TCP) cultures that include little or no ECM components. In addition to providing an increased capacity to bind and act as a reservoir for secreted proteins and other molecules, these substrates can also be designed to have a stress-to-strain ratio, or Young’s modulus, that approximates the “stiffness” of normal or fibrotic palmar fascia. The Young’s modulus of normal palmar fascia is lower than most tendons (Millesi et al. 1995) and approximates that of the dermis (10–1,000 Pa) (Hinz 2010; Yeung et al. 2005). Fibroblasts begin incorporating αSMA into their stress fibers, indicating their transition from fibroblasts to myofibroblasts, when ECM stiffness approaches 16,000–20,000 Pa (Hinz 2010). Tissues need to achieve a stiffness range of 25,000–50,000 Pa to maintain myofibroblasts in their hyper-contractile state (Hinz 2009). Interestingly, fibroblasts and myofibroblasts can respond to localized changes in the stiffness in their surroundings by migrating toward areas of increased stiffness through a process called durotaxis (Lo et al. 2000; Lange and Fabry 2013). Whether durotaxis contributes to the increased numbers of myofibroblasts in nodules or contracture cords is currently unknown.
TCP has a stiffness of at least 1,000,000,000 Pa (>1 gPa) (Achterberg et al. 2014), which is several orders of magnitude greater than the stiffness of any fibrotic tissue that fibroblasts or myofibroblasts could ever encounter in vivo. Under these conditions, fibroblasts spontaneously and robustly transition into αSMA-positive myofibroblasts without the need for any additional treatment interventions (Hinz et al. 2001). While there are applications where comparisons between uniform cultures of myofibroblasts are useful, it should nonetheless be appreciated that the behaviors or responses of cells under these conditions might have little or no similarity to their behaviors or responses on the substrates they normally interact with in vivo.
6.7 Interactions Between the Wnt/ß-Catenin Signaling Pathway and the Extracellular Matrix
This point can be illustrated by observing the interactions between Dupuytren Disease fibroblasts and myofibroblasts, cell culture substrates, and the Wnt/ß-catenin signaling pathway. Wnt signaling regulates ß-catenin levels during embryonic development and in a variety of diseases characterized by excessive cellular proliferation (Thompson and Monga 2007; Bowley et al. 2007; Manolagas and Almeida 2007). In the absence of Wnt signaling, ß-catenin is constitutively phosphorylated by casein kinase 1 and glycogen synthase kinase 3ß (GSK3ß), incorporated into a “destruction complex” that includes adenomatous polyposis coli (APC) and axin and degraded through the 26S proteasome (Bowley et al. 2007; Lam and Gottardi 2011). Wnt signaling induces the phosphorylation and inactivation of GSK3ß, thereby allowing ß-catenin to escape the destruction complex, accumulate in the cytoplasm, and translocate to the nucleus. Once in the nucleus, ß-catenin can bind transcription factors and act as a trans-activating factor to regulate gene expression (Bowley et al. 2007) (illustrated in Fig. 6.2).


Fig. 6.2
Wnt signaling regulates β-catenin levels. In the absence of wnt signaling (left), casein kinase 1 (CK1) and glycogen synthase kinase-3β (GSK-3β) phosphorylate β-catenin on serine/threonine residues, causing it to be sequestered to a “destruction complex” that includes adenomatous polyposis coli (APC) and axin, and its degradation through the 26S proteasome. Wnt signaling from the extracellular environment through the “canonical” frizzled receptor/low density lipoprotein receptor-related protein 5/6 (LRP5/6) pathway (right) results in phosphorylation of disheveled (Dvl), which directly or indirectly (through GSK-3β binding protein, GBP) phosphorylates and inactivates GSK-3β. GSK-3β inactivation allows β-catenin to avoid the destruction complex, accumulate within the cytoplasm, translocate to the nucleus, and trans-activate the transcription of genes associated with Dupuytren Disease development
The discovery of increased levels of ß-catenin in Dupuytren Disease tissues and in primary fibroblasts derived from these tissues (Varallo et al. 2003; Howard et al. 2003) led to the hypothesis that the Wnt/ß-catenin signaling pathway contributed to the pathogenesis of Dupuytren Disease. This hypothesis received indirect support when genome-wide association studies of patients with Dupuytren Disease identified single nucleotide polymorphisms (SNPs) in loci containing genes that encode Wnts or Wnt signaling-associated proteins (Dolmans et al. 2011). These findings led to a more detailed version of the original hypothesis that heritable abnormalities in Wnt gene expression result in dysregulated Wnt signaling, ß-catenin accumulation, and the development of Dupuytren Disease. While it is unclear if Wnt expression is dysregulated in Dupuytren Disease-derived fibroblasts or myofibroblasts (O’Gorman et al. 2006), these SNP-associated changes may impact transcriptional responsiveness to biochemical or biomechanical stimuli, gene transcript stability, or enhanced interactions with other pathways that may “cross-talk” with the Wnt/ß-catenin pathway, such as TNFα signaling (Verjee et al. 2013).
In addition to the potential effects of SNPs in or near Wnt or Wnt-related genes, the Dupuytren Disease ECM can independently regulate ß-catenin levels in myofibroblasts. While ß-catenin levels are clearly increased in contracture tissues relative to the levels in syngeneic (genetically identical) fibroblasts in adjacent, macroscopically unaffected palmar fascia (Howard et al. 2003; Varallo et al. 2003), these levels are rapidly normalized and become indistinguishable from those in cells derived from macroscopically unaffected palmar fascia when cultured from explant tissues onto TCP (Varallo et al. 2003). Transferring these cells from TCP into three-dimensional collagen-based cultures under isometric tension in fibroblast-populated collagen lattice assays restores the increased levels of ß-catenin; however, a rapid depletion of ß-catenin levels is evident once that tension is released (Varallo et al. 2003). In contrast, the dynamic regulation of ß-catenin levels in Dupuytren Disease myofibroblasts and ß-catenin levels in syngeneic myofibroblasts derived from macroscopically unaffected palmar fascia remain relatively stable under these conditions (Varallo et al. 2003). When cultured in low-density type-1 collagen substrates with little or no isometric tension, ß-catenin levels in myofibroblasts derived from contracture tissues are depleted over 72 h until they are virtually undetectable by immunoblotting (Vi, Njarlangattil, et al. 2009). While these culture conditions also induce some depletion of ß-catenin levels in syngeneic myofibroblasts derived from macroscopically unaffected palmar fascia, the effects are modest and variable between cultures (Vi et al. 2009b). TGFβ-1, the ECM-associated cytokine that is well known to promote the development of myofibroblasts (Badalamente et al. 1996), restores ß-catenin levels in Dupuytren Disease myofibroblasts cultured in low-density type-1 collagen substrates (Vi et al. 2009b). While it is currently unclear whether matrix stiffness, collagen signaling, or both are required to elicit these effects on ß-catenin levels in Dupuytren Disease myofibroblasts, it is clear that these cells are abnormally sensitive to these factors relative to syngeneic myofibroblasts derived from macroscopically unaffected palmar fascia.
These findings predict that the outcomes of Wnt/ß-catenin signaling analyses in Dupuytren Disease will be dependent on the culture substrates used during the analyses. Such effects are predicted to extend beyond the expression of genes that are trans-activated by ß-catenin and translated into fibrosis-associated proteins and may also include the cytokines and ECM-associated signaling molecules that act in parallel to enhance or attenuate Wnt/ß-catenin signaling. Thus, before we perform in vitro analyses of therapeutic interventions that modify Wnt/ß-catenin signaling in Dupuytren Disease, it is essential that we make informed choices regarding the culture substrates in which such analyses take place (Fig. 6.3).


Fig. 6.3
The extracellular matrix regulates β-catenin levels. Many different cytokines, including transforming growth factor-β (TGFβ) and tumor necrosis factor α (TNFα), can signal through pathways that intersect with the Wnt/β-catenin signaling pathway and increase intracellular β-catenin levels. While the mechanisms are currently unclear, extracellular matrix (ECM) factors, such as collagen density, can increase or decrease intracellular β-catenin levels in Dupuytren Disease myofibroblasts, and thereby potentially modify β-catenin signaling in parallel with cytokine-activated pathways. As such, the potential confounding influences of the Dupuytren Disease ECM should be taken into account when assessing the effects of therapeutic interventions designed to modify cytokine or other signaling pathways that regulate β-catenin signaling in Dupuytren Disease
6.8 Targeting the Extracellular Matrix to Attenuate Dupuytren Disease Development
If we accept that the ECM surrounding Dupuytren Disease myofibroblasts modifies their responses and actively promotes disease progression, then the ECM itself can be considered as a therapeutic target. The concept of targeting the ECM in Dupuytren Disease is not new. J. T. Hueston, one of the “founding fathers” of Dupuytren Disease research, originally suggested that “enzymic fasciotomy” (Hueston 1971) could achieve similar outcomes to surgical fasciotomy in select patients. His approach was to use a cocktail of proteolytic enzymes and anti-inflammatory agents to degrade the ECM-associated collagens in contracture cords while simultaneously dampening the effects of pro-fibrotic inflammatory cytokines. While Hueston’s approach did not gain broad acceptance at the time, targeting the ECM-associated collagens in contracture cords has now become a therapeutic reality. Xiaflex/Xiapex® (Hurst and Badalamente 1999; Badalamente et al. 2002; Hurst et al. 2009) is a mixture of Clostridium histolyticum type I and type II collagenases that specifically target the amino- and carboxy-termini and internal peptide residues of the type I and type III collagens in the Dupuytren Disease ECM. While this approach has many advantages over more invasive treatment options, it is worth noting that degradation of type I and type III collagens in the ECM, while effective for restoring hand function in the short term, is insufficient to prevent Dupuytren Disease recurrence (Watt et al. 2012; Baltzer and Binhammer 2013; Chen et al. 2011). The consequences of disrupting the biochemical and biomechanical signals that myofibroblasts receive from their collagen-enriched ECM under tension remain poorly understood at the molecular level and worthy of detailed investigation. Controlled proteolysis of collagens and other ECM proteins can generate bioactive molecules known as matricryptins (Ricard-Blum and Ballut 2011) that stimulate a wide variety of cellular responses including proliferation, migration, and angiogenesis (Ricard-Blum and Salza 2014). It is currently unclear whether matricryptins or other biologically active factors derived from ECM degradation contribute to Dupuytren Disease recurrence after Xiaflex/Xiapex® treatments.
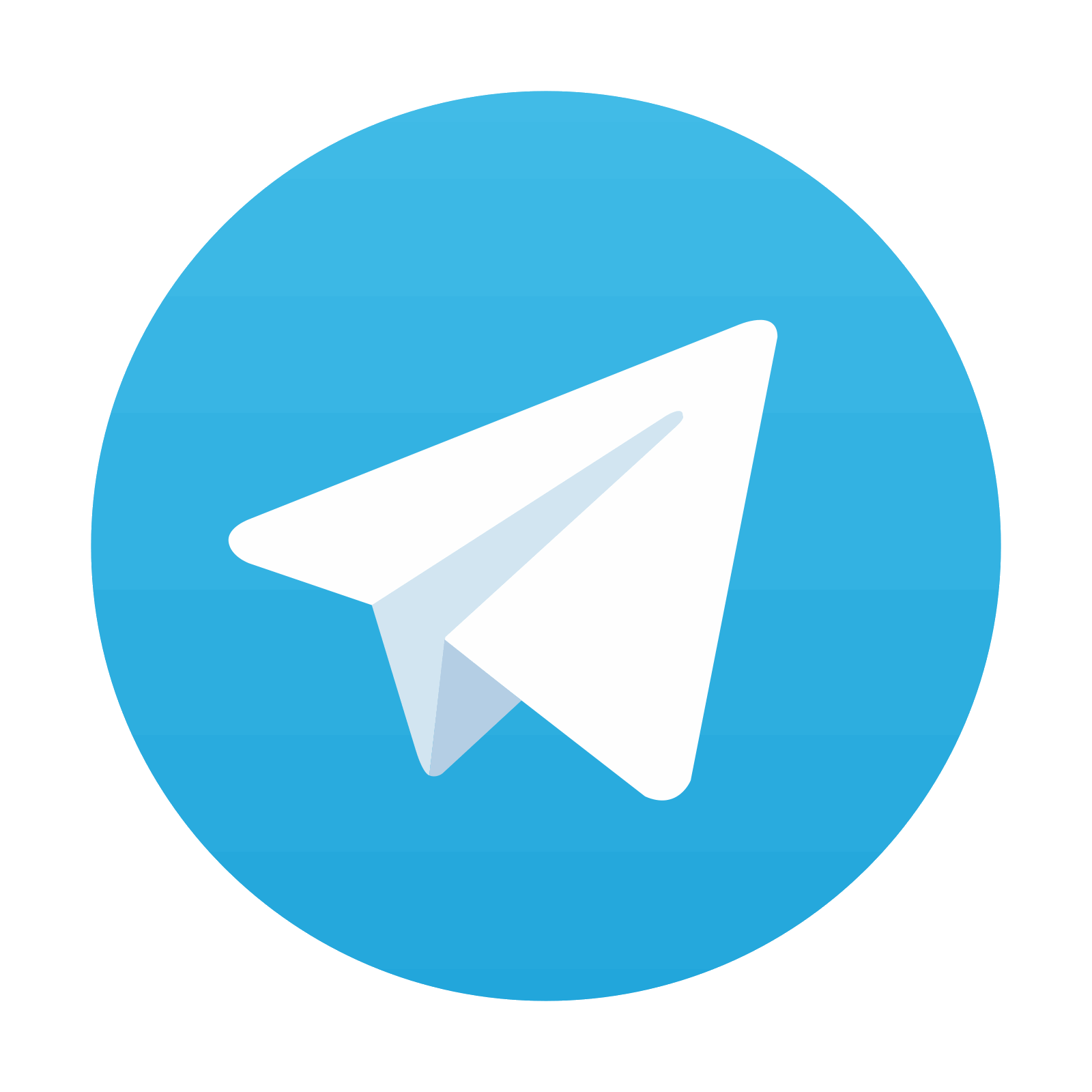
Stay updated, free articles. Join our Telegram channel
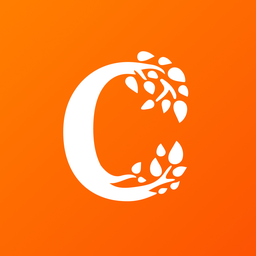
Full access? Get Clinical Tree
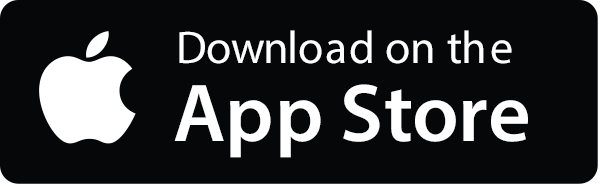
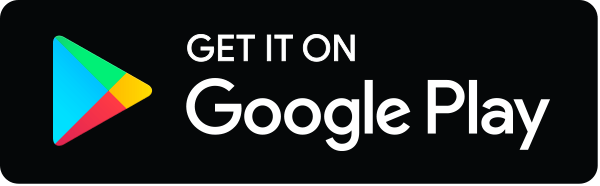