The Development, Growth, and Morphogenesis of the Skeleton
Maurizio Pacifici, PhD
Eiki Koyama, DDM, PhD
Neither of the following authors nor any immediate family member has received anything of value from or has stock or stock options held in a commercial company or institution related directly or indirectly to the subject of this chapter: Dr. Pacifici and Dr. Koyama.
Keywords: growth plate; joint formation; morphogenesis; pediatric skeletal disorders; skeletal development
INTRODUCTION
The skeleton can be thought of as the ultimate morphogenetic machine determining the overall shape, size, organization, and features of the body. Paleontologists and biological anthropologists have long used bone fragments to envision and reconstruct the anatomic features of distant human ancestors, down to the level of facial composition and expression.1 Thus, the formation, sculpting, and growth of the skeletal elements must be regarded as a feat of development, structure, organization, and evolution.2 Biomedical research over recent decades has provided many important data and insights into mechanisms by which the skeletal tissues form and grow during fetal and postnatal life, how they develop their organization and structure, and how the major skeletal cell types (chondrocytes, osteoblasts, osteocytes, and osteoclasts) acquire their diversified and essential phenotypic functions.3,4,5,6,7,8 Most skeletal elements, including the long bones, ribs, vertebrae, cranial base, vertebrae, and pelvis, are formed through endochondral ossification. During this process, mesenchymal cells differentiate into chondrocytes that produce and assemble the initial cartilaginous skeletal primordia and templates at prescribed sites and times, which serve as the blueprint for the corresponding ossified elements in the adult skeleton. The chondrocytes become organized into growth plates that represent the major engine of skeletal expansion and elongation. In the growth plates, the chondrocytes proliferate, accumulate large amounts of extracellular matrix, and undergo hypertrophy. The hypertrophic chondrocytes mineralize their matrix and provide the mineralized scaffold onto which osteoprogenitor cells differentiate and produce endochondral bone tissue.6 The remaining skeletal elements including the calvaria, mandible, and a portion of the clavicle form through intramembranous ossification.9 Ectomesenchymal cells differentiate directly into bone cells during this process.
Though distinct in many respects, the endochondral and intramembranous processes are more intimately connected than might be apparent. The first bone tissue to form in a developing long bone is intramembranous and is called the bone collar because it surrounds the incipient diaphysis. Likewise, the mandible forms in intimate association with the Meckel cartilage. The mandibular condyle, which articulates with the glenoid fossa to form the temporomandibular joint, is entirely endochondral. Research has also revealed that cells similar to chondrocytes are transiently present during the early stages of calvaria bone formation.10
In sum, cartilage and bone form and work in concert at multiple places and times during prenatal and postnatal life to bring about skeletogenesis and skeletal growth and morphogenesis. This chapter describes the key steps in the formation of the craniofacial, axial, and appendicular skeleton and discusses recent data on mechanisms regulating the behavior and function of skeletal progenitor and differentiated cells and the growth of skeletal elements. It also describes salient examples
of defects in such basic growth and developmental mechanisms that lead to congenital and acquired skeletal conditions in children and adults.
of defects in such basic growth and developmental mechanisms that lead to congenital and acquired skeletal conditions in children and adults.
CRANIOFACIAL DEVELOPMENT AND GROWTH
The progenitor cells responsible for formation of the craniofacial skeletal elements originate embryonically from the ectodermal neural crest, the unsegmented paraxial mesoderm, and the most cephalic somites.11,12 Between the first and second month of gestation, the progenitors migrate from their points of origin, proliferate, and assemble to form cell condensations at appropriate sites, producing initially over 100 distinct skeletogenic centers in the prospective skull.12 Over increasing gestation time, consolidation and fusion amongst these skeletal primordial centers elicit formation of 45 skeletal elements by birth. Postnatal processes bring about a final reduction in number and the assembly of the mature 22 bones in the adult skull.
The different skull skeletal elements brought about by the above processes arise via an intramembranous or endochondral ossification process. The intramembranous elements (also called dermal bones) include the frontal and parietal cranial vault bones, the squama of the occipital and temporal bones, the zygomatic bone, the maxilla, and the mandible (Figure 1). The endochondral elements include the occipital bone, the cranial base, the mandibular condyle, the auditory ossicles, and the nasal bones (Figure 1). An alternative and common classification of skull skeletal elements takes into account their function rather than origin. Thus, the neurocranium includes the elements that surround and protect the brain—cranial vault and cranial base—and the sensory organs. The viscerocranium includes the elements of the face and pharyngeal arches.
Three-dimensional growth of the intramembranous elements continues through the remaining months of gestation and into early childhood. The process occurs mostly by apposition, during which progenitor cells differentiate into osteoblasts and are apposed onto the preexisting bone tissue. The progenitor cells responsible for appositional growth of the cranial vault elements are part of the fibrous connective and mesenchymal tissue masses called sutures and are connected at the fontanelles located at the corners where the elements meet (Figure 1). Progenitor cell function and differentiation in the sutures are controlled by various mechanisms. Chief among these mechanisms is action by members of the fibroblast growth factor (FGF) and hedgehog families of signaling proteins that may also be important in dictating progenitor cell function during skull fracture repair.13,14 Growth and expansion of the cranial vault are closely coordinated with growth and expansion of the brain. The posterior and anterolateral fontanelles close during the first 3 months after birth; the other fontanelles remain open and close at approximately the age of 2 years. Defects and discoordination of these processes can have serious pathological consequences (see below).
The growth plates are responsible for enlargement and elongation of the craniofacial endochondral elements. Because the growth plates can be oriented in different directions and vary in size, they support and regulate skeletal elongation and ossification in distinct spatiotemporal directions and can fine-tune morphogenesis in three dimensions. One example is the exquisite shape and organization of the sella turcica of the sphenoid bone, which accommodates the pituitary gland. A special group of growth plates is present in the cranial base that is composed of the ethmoid, sphenoid, and occipital bones and other elements. These growth plates are called synchondroses, are active during fetal and early postnatal life, and are named according to their anatomical location: intrasphenoidal, spheno-occipital, and intraoccipital synchondroses (Figure 2, A and B). Each synchondrosis consists of two opposing, mirror-image growth plates that share a central reserve chondrocyte zone, display two proliferative, two prehypertrophic, and two hypertrophic zones, and sustain bidirectional growth along the naso-occipital axis (Figure 2, C and D). Despite their unique dual structure, the synchondrosis growth plates are regulated by most of the mechanisms that control the activity of single growth
plates in all other locations in the developing and growing skeleton.15,16 Under normal circumstances, the intraoccipital synchondrosis closes at the age of 1 to 3 years, while the spheno-occipital synchondrosis closes at puberty.
plates in all other locations in the developing and growing skeleton.15,16 Under normal circumstances, the intraoccipital synchondrosis closes at the age of 1 to 3 years, while the spheno-occipital synchondrosis closes at puberty.
Clinically, defects in suture growth function and/or timing of closure can cause craniosynostoses that affect cranial base elongation and growth coordination and often have severe pathologic repercussions on brain development. Most craniosynostosis cases are due to gain-of-function mutations in the FGF receptor genes FGFR1, FGFR2, or FGFR3 that alter the fine physiologic balance between proliferation and differentiation of progenitor cells, cause excessive bone differentiation, and provoke dysfunction and premature closure of the sutures.17,18 Craniosynostoses affecting specifically the coronal suture are seen in patients with mutations in the transcription factor TWIST or the membrane-bound protein EPHRIN-B1. One side of the coronal suture arises from neural crest progenitors and the opposite side arises from mesodermal cells, and the boundary appears to be particularly sensitive to those mutations.19 Defects in synchondrosis growth plate function and elongation rates can cause hypoplasia of the cranial base, as seen in Apert and Crouzon syndromes, and can have negative repercussions on overall skull growth, structure and organization.20 Holoprosencephaly is a devastating condition caused by loss-of-function mutations in genes encoding Sonic hedgehog (SHH) or its receptor PATCHED1.21 Hedgehog signaling is mediated by primary cilia on the surface of most cell types, and human syndromes affecting the craniofacial skeleton and other organs, such as Meckel-Gruber syndrome, are caused by malfunction of primary cilia-associated proteins.22
SOMITOGENESIS AND AXIAL SKELETON DEVELOPMENT
Beginning at approximately the third week of gestation, mesodermal cells flanking the neural tube and notochord (collectively called paraxial mesoderm) begin to locally condense, undergo a mesenchymal-to-epithelial transition
(MET), and produce the first pair of somites near the incipient anatomical border between the future head and the trunk (Figure 3, A, left panel).23 Additional pairs of somites form by the same process at very regular, short intervals along the cranial-to-sacral axis, a process known as the “segmentation clock.” Somitogenesis is completed in approximately 1 week with the formation of more than 40 pairs of somites located all along the left and right sides of the notochord and neural tube. Rhythmic formation of each somite pair is driven by rapid cycles of activity by—and molecular integration among—three major signaling pathways: Notch, Wnt/β-catenin, and FGF.24 The remarkable precision of the segmentation clock is exemplified by formation of a new somite pair every 90 minutes in chick embryos and every 20 minutes in zebrafish embryos.25 Soon thereafter, the cells constituting the ventral portion of each somite pair (collectively known as the sclerotome) undergo an inverse process of epithelial-to-mesenchymal transition (EMT) and migrate toward and gather around the notochord, which remains located immediately below the neural tube (Figure 3, A, middle panel). Sonic hedgehog secreted by both the notochord and the adjacent ventral portion of the neural tube creates a concentration gradient in its surroundings and is responsible for such directional migration of the sclerotomal cells. It should be noted that cells constituting the dorsal portion of each somite are distinct and represent the dermomyotome (Figure 3, A, right panel) that will give rise to such tissues as the dermis and skeletal muscles in the trunk and limbs. The relatively few cells sandwiched between the dermomyotome and the sclerotome become marked by scleraxis expression, serve as progenitors for axial tendon formation and are together known as the syndetome (Figure 3, A, right panel).26
(MET), and produce the first pair of somites near the incipient anatomical border between the future head and the trunk (Figure 3, A, left panel).23 Additional pairs of somites form by the same process at very regular, short intervals along the cranial-to-sacral axis, a process known as the “segmentation clock.” Somitogenesis is completed in approximately 1 week with the formation of more than 40 pairs of somites located all along the left and right sides of the notochord and neural tube. Rhythmic formation of each somite pair is driven by rapid cycles of activity by—and molecular integration among—three major signaling pathways: Notch, Wnt/β-catenin, and FGF.24 The remarkable precision of the segmentation clock is exemplified by formation of a new somite pair every 90 minutes in chick embryos and every 20 minutes in zebrafish embryos.25 Soon thereafter, the cells constituting the ventral portion of each somite pair (collectively known as the sclerotome) undergo an inverse process of epithelial-to-mesenchymal transition (EMT) and migrate toward and gather around the notochord, which remains located immediately below the neural tube (Figure 3, A, middle panel). Sonic hedgehog secreted by both the notochord and the adjacent ventral portion of the neural tube creates a concentration gradient in its surroundings and is responsible for such directional migration of the sclerotomal cells. It should be noted that cells constituting the dorsal portion of each somite are distinct and represent the dermomyotome (Figure 3, A, right panel) that will give rise to such tissues as the dermis and skeletal muscles in the trunk and limbs. The relatively few cells sandwiched between the dermomyotome and the sclerotome become marked by scleraxis expression, serve as progenitors for axial tendon formation and are together known as the syndetome (Figure 3, A, right panel).26
The sclerotomal cells gathering around the notochord and neural tube are responsible for generating the vertebrae and eventually, the ribs. In terms of vertebral developmental steps, the sclerotomal cells from each somite first become phenotypically subdivided into a larger cranial portion and a smaller caudal portion, and the border between them is called the intersegmental boundary or von Ebner fissure (Figure 3, B). Cranial cells from one somite pair intermix and condense with the caudal cells from the preceding somite pair and together, will produce each vertebra (Figure 3, B). Mixing and condensation of these subpopulations are regulated by expression of the ephrin receptors EPHA4 and EPHB3 in cranial cells and expression of their receptor ligand EPHRIN-B1 in caudal cells.27 The intersegmental
boundary within each somite represents the location where the intervertebral disk forms (Figure 3, B) and where the spinal nerves leave the spinal cord to innervate their diverse peripheral targets. To produce each vertebra, the condensed sclerotomal cells first undergo chondrogenesis and produce three-dimensionally complex cartilaginous templates corresponding to the distinct structure and portions of each vertebra, including the vertebral body, neural arches around the future vertebral foramen, and transverse processes. The notochordal cells trapped within the developing vertebral body undergo involution and disappear. The newly formed chondrocytes become organized into distinct growth plates, undergo hypertrophy and endochondral ossification, and produce the definitive bony vertebrae that surround the spinal cord. Spared from these maturation and hypertrophic processes are the chondrocytes located at the cranial and caudal vertebral ends that remain cartilaginous and produce the end plates articulating with the intervertebral disks (see below). As pointed out above, more than 40 somite pairs form initially.23 However, the initial sclerotomes from the four occipital somites and the first cervical sclerotome aggregate to produce parachordal cartilages which fuse at midline and produce the occipital bone of the skull. In addition, many pairs of sacral somitic sclerotomes fuse to produce the sacrum and the coccyx, eventually leading to the establishment of the definitive 24 vertebrae. As these developmental and growth processes proceed, the spine acquires its typical species-specific curvature, as required for its normal biomechanical function and movement.28 Very little is known of the mechanisms that regulate the spatiotemporal acquisition of normal spine curvature.
boundary within each somite represents the location where the intervertebral disk forms (Figure 3, B) and where the spinal nerves leave the spinal cord to innervate their diverse peripheral targets. To produce each vertebra, the condensed sclerotomal cells first undergo chondrogenesis and produce three-dimensionally complex cartilaginous templates corresponding to the distinct structure and portions of each vertebra, including the vertebral body, neural arches around the future vertebral foramen, and transverse processes. The notochordal cells trapped within the developing vertebral body undergo involution and disappear. The newly formed chondrocytes become organized into distinct growth plates, undergo hypertrophy and endochondral ossification, and produce the definitive bony vertebrae that surround the spinal cord. Spared from these maturation and hypertrophic processes are the chondrocytes located at the cranial and caudal vertebral ends that remain cartilaginous and produce the end plates articulating with the intervertebral disks (see below). As pointed out above, more than 40 somite pairs form initially.23 However, the initial sclerotomes from the four occipital somites and the first cervical sclerotome aggregate to produce parachordal cartilages which fuse at midline and produce the occipital bone of the skull. In addition, many pairs of sacral somitic sclerotomes fuse to produce the sacrum and the coccyx, eventually leading to the establishment of the definitive 24 vertebrae. As these developmental and growth processes proceed, the spine acquires its typical species-specific curvature, as required for its normal biomechanical function and movement.28 Very little is known of the mechanisms that regulate the spatiotemporal acquisition of normal spine curvature.
The intervertebral disks are critical for spine movement and shock absorption function. Each disk is composed of a centrally located nucleus pulposus and a surrounding anulus fibrosus (Figure 4, A). These two components have distinct embryologic origin. The progenitor cells producing the anulus fibrosus are those sclerotome cells located along the intersegmental boundary that do not participate in vertebra formation. The cells are still gathered around the notochord, but undergo differentiation into fibrocartilaginous cells that produce and deposit a matrix rich in collagens I and II endowed with stiffness and tensile strength, all needed for proper biomechanical function by the annulus. Instead, the progenitor cells responsible for nucleus pulposus formation are notochord cells, specifically those that become surrounded by intersegmental sclerotome cells.29 Unlike the ephemeral notochord cells within each developing vertebral body, these notochord cells persist, differentiate into chondrocyte-like cells, and produce and accumulate a large amount of cartilaginous extracellular matrix. This matrix is rich in aggrecan and other proteoglycans but poor in collagen; it attracts a large quantity of water and ions and establishes a high osmotic pressure, which is believed to be essential for establishing the functional springiness and resilience of the disk. The intervertebral disk (anulus fibrosus plus nucleus pulposus) and flanking vertebral end plates together make up the joints of the spine, which, similar to synovial joints in the limbs, are characterized by local production of lubricating molecules such as lubricin (proteoglycan 4) that sustain movement and functional longevity and minimize mechanical friction (Figure 4, B and C).
With regard to the ribs, their development initiates at approximately the fifth week of embryogenesis with formation of small protrusions of lateral sclerotome-derived mesenchymal cells along the arches of all developing vertebrae. Only those in the thoracic region continue to grow and with time, curve ventrally to provide appropriate directionality to the mature ribs. The elongating thoracic ribs reach the developing sternum by embryonic day 45. Five additional lumbar ribs form that, however, are short, do not articulate with the sternum, and are called false ribs. All ribs are endochondral elements; they develop and elongate through the activities of a single growth plate that progressively shifts in relative position from dorsal toward ventral over time. The most ventral tip of the true ribs maintains a cartilaginous character to provide articulation with the sternum.
Clinically, a number of common and rare disorders affect the axial skeleton, not surprising given the many demanding functions it performs and the complex mechanisms regulating both its development and homeostasis. For example, degeneration of the intervertebral disks and consequent back, neck, and radicular pain remain major health challenges for a large fraction of the population, particularly the elderly.30 These conditions are associated with progressive derangement of disk structural and phenotypic integrity, including loss of extracellular proteoglycan matrix and water, thinning and stiffening of the tissues, and, ultimately, loss of functional resilience. Major pathogenic mechanisms include death of notochord-derived nucleus pulposus cells by apoptosis; increased levels of proinflammatory cytokines and immune cell infiltration; and ectopic appearance of microvessels and nerve fibers.31 Current therapeutic attempts include stem cell therapies with notochord-like progenitors, surgical implantation of bioengineered disks, and antagonism of cytokine function.32 One example of rare spine disorders are congenital forms of scoliosis that can be very pernicious, encompass several phenotypic patterns, and are difficult to treat.33 Their pathogenesis includes generalized abnormalities in fetal vertebral segmentation and rib development as seen in patients with spondylothoracic dysostosis; regional abnormalities affecting a portion of the spine, such as cervical defects in Klippel-Feil patients; and local abnormalities affecting one or two vertebrae. Identification of siblings with congenital scoliosis has led to identification of possible causative mutations in genes that, interestingly, regulate the somatic segmentation clock and include such Notch signaling pathway components as DLL3, HES7, and LUNATIC FRINGE.24
LIMB PATTERNING AND ONSET OF SKELETOGENESIS
At approximately 3 weeks of embryogenesis, small protrusions known as limb buds begin to form along the incipient trunk at prescribed locations and represent the first overt sign of forelimb (arm) and hindlimb (leg) development. The limb buds are composed of an ectodermal tissue layer and are filled with mesenchymal cells. The latter originate from the lateral mesoderm, the somitic dermomyotome, and the neural crest,12 and this triple origin reflects their distinct developmental fates and contributions over time. Thus, lateral mesoderm cells will produce all the limb skeletal elements, connective tissues, and ligaments; the somitic cells will produce all the limb skeletal muscles and endothelial cells; and the neural crest cells will produce melanocytes and Schwann cells. Once the limb buds appear, their further outgrowth progresses rapidly and is propelled and oriented by three key signaling centers acting along the proximal-distal, posterior-anterior, and dorsal-ventral axes of symmetry34,35 (Figure 5, A and B). The apical ectodermal ridge (AER) is located at the very tip of the limb bud and regulates outgrowth and patterning along the proximal-to-distal axis (Figure 5, A). The AER cells produce FGF4, FGF8, FGF9, and FGF17, which diffuse and stimulate proliferation of the adjacent mesenchymal cells (collectively known as the progress zone), resulting in limb outgrowth. Experimentally, limb elongation stops if the ectodermal ridge is removed, but it can resume with application of exogenous recombinant FGFs. The zone of polarizing activity (ZPA) regulates patterning along the posterior-anterior axis. The ZPA consists of mesenchymal cells specifically located at the posterior margin of each limb bud that produce sonic hedgehog (Figure 5, B). SHH diffuses away from the cells, forms a concentration gradient along the posterior-to-anterior axis, and regulates patterning and skeletal element identity. For example, the high posterior concentration of SHH favors small finger formation, and the low anterior concentration of SHH favors thumb/big toe development. If the ZPA is
microsurgically removed, the posterior-anterior axis of symmetry is completely disrupted. On the other hand, if an additional ZPA from a donor embryo is implanted into the anterior margin of a host limb bud (so that the host limb has two ZPAs and, thus, two opposite sources of SHH), two sets of limb skeletal elements form over time and are oriented in a mirror-image fashion along the posterior-to-anterior axis. Lastly, cells present in the dorsal ectoderm regulate limb patterning and skeletal growth along the dorsal-to-ventral axis and express factors such as wingless-type family member 7A (WNT7A) and homeobox protein LMX1B. When the dorsal ectoderm is microsurgically removed and transplanted to the ventral side, the dorsal-ventral axis is reversed 180° (eg, foot pads form on the dorsal side). A similar axis inversion is seen in transgenic Wnt7a-null mice. WNT7A function is mediated by LMX1B. Mice that are mutant for Lmx1b in the limbs lack dorsal structures; they also have defects along the posterior-anterior axis because Lmx1b regulates Shh expression in the ZPA.36
microsurgically removed, the posterior-anterior axis of symmetry is completely disrupted. On the other hand, if an additional ZPA from a donor embryo is implanted into the anterior margin of a host limb bud (so that the host limb has two ZPAs and, thus, two opposite sources of SHH), two sets of limb skeletal elements form over time and are oriented in a mirror-image fashion along the posterior-to-anterior axis. Lastly, cells present in the dorsal ectoderm regulate limb patterning and skeletal growth along the dorsal-to-ventral axis and express factors such as wingless-type family member 7A (WNT7A) and homeobox protein LMX1B. When the dorsal ectoderm is microsurgically removed and transplanted to the ventral side, the dorsal-ventral axis is reversed 180° (eg, foot pads form on the dorsal side). A similar axis inversion is seen in transgenic Wnt7a-null mice. WNT7A function is mediated by LMX1B. Mice that are mutant for Lmx1b in the limbs lack dorsal structures; they also have defects along the posterior-anterior axis because Lmx1b regulates Shh expression in the ZPA.36
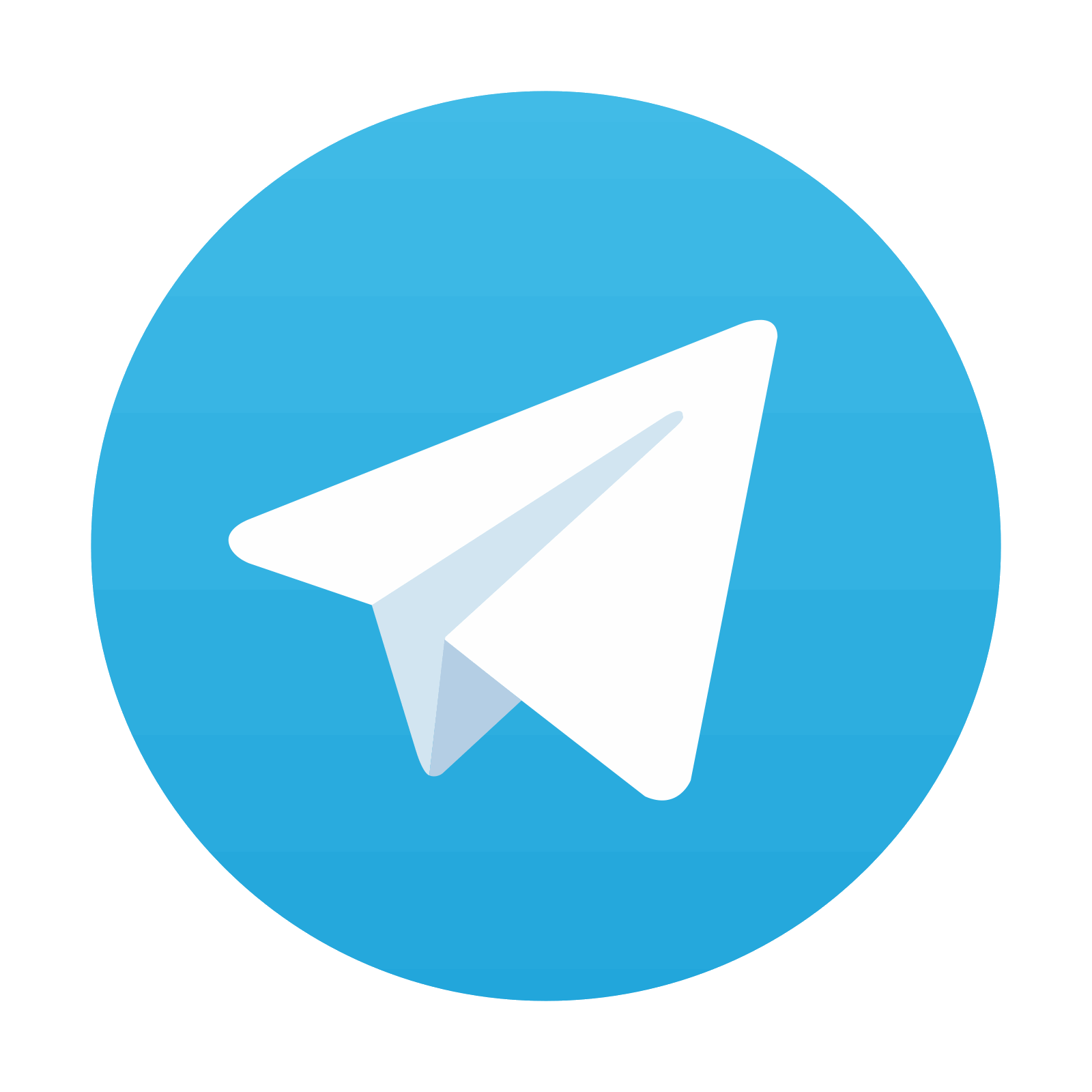
Stay updated, free articles. Join our Telegram channel
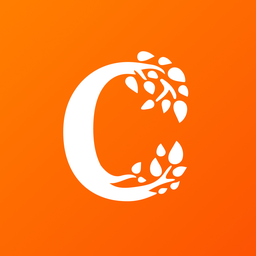
Full access? Get Clinical Tree
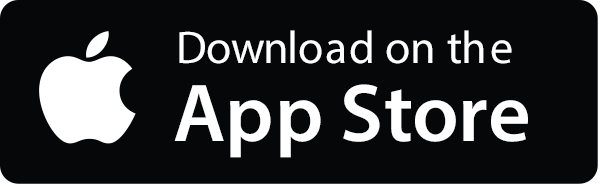
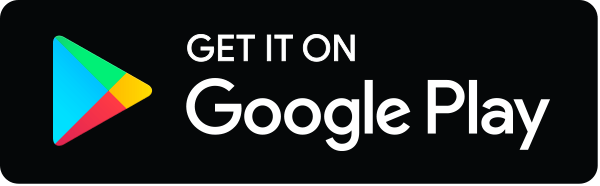