© Springer International Publishing Switzerland 2017
Norbert Weidner, Rüdiger Rupp and Keith E. Tansey (eds.)Neurological Aspects of Spinal Cord Injury10.1007/978-3-319-46293-6_2020. The Current Status of Neuroprotection for Spinal Cord Injury
(1)
The Miami Project to Cure Paralysis, University of Miami Miller School of Medicine, 1095 NW 14th Terrace, Miami, FL 33136, USA
(2)
The Miami Project to Cure Paralysis and Neurological Surgery, University of Miami Miller School of Medicine, 1095 NW 14th Terrace, Miami, FL 33136, USA
Abstract
Spinal cord injury (SCI) resulting in impaired neurological functions creates suffering and economic burden. Directly after the injury, the conservation of tissue at the lesion site and the preservation of the connectivity through the injury epicenter have highest priority. This tissue preservation will not only reduce neurological deficits, but also and allows for more rapid and extensive recovery. Advances in clinical care intended to accomplish this goal include early surgical decompression, support of blood pressure, and the subject of this chapter – experimental neuroprotective therapeutics. The scientific foundation of neuroprotection is that “harmful” secondary injury processes extend and distribute the tissue loss caused by the primary injury event. Existing clinical knowledge together with preclinical experimental evidence has supported phase III clinical trial translation of some neuroprotective agents in the past four decades. Although some have had positive results, the magnitude of improvement was small, and associated complications and controversy surrounding certain therapeutics diminished their role in SCI care. However, these trials generated knowledge valuable to guide current work. Neuroscientists continue to develop new therapeutic approaches by demonstrating neuroprotective efficacy in small and large animal models. A consensus now exists that the preclinical data set to support the expensive process of translation must be very robust and include a well conducted independent replication. Primary endpoints for pivotal clinical trials have been clarified on the basis of aggregate experience and extensive studies on the natural history of SCI. “Secondary injury” consists of numerous mechanisms, and the probability of a robust protective effect of a single agent is small. It is necessary to design rational combinations of therapies to increase efficacy.
20.1 Introduction
Scientists often have a naive faith that if only they could discover enough facts about a problem, these facts would somehow arrange themselves in a compelling and true solution. Theodosius Dobzhansky
The capability of central nervous system (CNS) tissue for self-repair after severe injury is often insufficient to restore important neurological functions. Due to its small size and axonal concentration, the spinal cord is especially vulnerable to traumatic damage. The primary injury to the spinal cord results from an abrupt physical distortion such as occurs with a diving accident or a military blast injury. Cascades of molecular, cellular, tissue, and organ level events, many of which are pathophysiological, are initiated and propagate the tissue loss caused by the instantaneous primary injury. These events, collectively known as “secondary injury,” are thought to develop in proportion to the primary insult with a consequent progressive loss of residual gray and white matter around the injury epicenter. Secondary injury starts immediately, and its duration depends on which mechanism of pathophysiology is considered (e.g., excitotoxicity, ionic dysregulation, free radical toxicity and lipid peroxidation, mitochondrial and membrane failure, axonal disintegration, apoptosis and cell death, demyelination, inflammation) (see chapter 19), but can last at least weeks. The secondary injury concept is a generalization whose value may be superseded as more specific knowledge of post-injury processes is developed. Neuroprotection is also a broad concept applied to many types of neural injury referring to the attenuation of impending injury. The possibility to provide neuroprotection has been proven in certain settings, e.g., tissue cooling increases the brain and spinal cord’s tolerance to ischemia when initiated prophylactically [34, 267]. In these scenarios, tissue cooling neuroprotects the CNS during ischemia that would otherwise cause neuronal death.
However, more commonly neuroprotection refers to reduction of damage that would otherwise occur after an unpredictable event such as traumatic SCI. The development and testing of neuroprotective agents has largely followed emerging knowledge of pathomechanisms of secondary injury. Thus, early efforts focused on problems such as lowered spinal cord blood flow (SCBF) for which experimental evaluation techniques existed [272]. Current efforts are directed to control molecular events for which newer biomarker measurements are available.
The CNS has unique tissue properties, responses, and vulnerability; neuronal cells extend processes for long distances with high complexity and specificity. Neurons have high metabolic requirements and must maintain membrane function within narrow parameters. CNS glia also have complex functions including regulation of neurotransmitter levels and formation of myelin. The blood-brain barrier prevents the crossing of many classes of drugs, cells, proteins, and other molecules from the blood into the CNS extracellular space. Thus, specialized pharmacokinetic and pharmacodynamic studies are necessary to understand delivery and distribution to the brain and spinal cord. These include the administration route, bioavailability, serum, CSF and tissue levels, half-life, route of elimination, and metabolism to active and inactive products, including the impact of focal blood-brain barrier disruption. Critical issues such as a therapeutic window, dose-response, and adverse or secondary effects must be determined in preclinical animal models and phase I/II human studies. Neuroprotectants range from physical processes that affect the entire body (hypothermia) to drugs, ions, and specially engineered proteins and other biomolecules.
There has been a high failure rate of neuroprotective therapies applied clinically in stroke, traumatic brain injury, and SCI despite many impressive experimental studies in animals. To understand the reasons for this, and to increase efficiency and effectiveness, emphasis has been directed toward both the need for greater rigor in preclinical testing and clinical trial design [123, 196], increased reporting of negative results [229, 304], and the development of quantitative biomarkers.
Another issue to consider is that the distinction between primary and secondary injury is artificial because much of what is called secondary injury is directly triggered by the primary insult, as is a bruise following a blow to an extremity. Thus, the extent to which the consequences of primary injury can be attenuated may be limited. Another key distinction is between secondary injury and secondary insult, the latter being, e.g., an additive insult influenced by therapeutic management such as prolonged hypotension or by concomitant injuries such as hypoxia from pulmonary injuries. This chapter will summarize current knowledge of neuroprotective strategies in SCI discussing the major lessons so far learned and prospects for the near future.
20.2 Non-pharmacological Strategies for Neuroprotection
20.2.1 Surgical Decompression
The relief of ongoing pressure on the spinal cord and stabilization of the spine are indicated after SCI when either significant compression or instability is present. Decompression is the most direct neuroprotective procedure. Without spinal cord decompression, many other neuroprotective strategies are futile. If spinal cord compression raises the tissue pressure, ischemia may compromise tissue that might otherwise survive [46, 78]. Early decompression after SCI was assessed in several rodent models. These have shown improved behavioral and anatomical outcomes when decompression was performed earlier, e.g., 6 and even 12 h post-SCI, rather than 24 [284]. There may also be a role for adding dural enlargement to spinal cord decompression. Four-hour post-injury durotomy with allogeneic duraplasty reduced spinal cord cavitation, inflammation, and scar formation when compared to injury or durotomy only control animals [293]. A recent clinical study showed that following SCI, duraplasty improved estimated spinal cord perfusion more than spinal laminectomy decompression alone [248]. Recently, even the acute decompression of necrotic spinal cord tissue has been assessed as a neuroprotectant in large animal models, thus the definition of effective spinal decompression after SCI is in evolution.
Although evidence in animal models shows a benefit to early spinal cord decompression, the optimal clinical timing has been a leading controversy in neurosurgery in recent decades and remains without conclusive evidence. Concerns regarding early surgery have included the safety of acute surgical manipulation of the spinal cord; safety of transportation to specialized care centers; availability of skilled spine surgeons, anesthesiologists, and operative teams at all hours; availability of imaging; and vulnerability of acutely damaged spinal tissue to fluctuations in blood pressure during anesthesia. Substantial published evidence has addressed these concerns supporting the safety of early surgery [101, 112, 134, 331]. The spine surgery culture has shifted to recognize the value of early surgery. An evaluation of the clinical effectiveness of decompressive surgery after SCI took place with the STASCIS Study (Surgical Timing in Acute Spinal Cord Injury). This multicenter, international, prospective, nonrandomized study analyzed the effects of early (<24 h post-SCI) versus late decompression (≥24 h post-SCI) [101]. From 313 patients enrolled, 222 were assessed at the 6-month post-SCI interval; 19.8 % of the early surgery cohort showed a ≥2 grade improvement in AIS grade versus 8.8 % in the late group. There were some imbalances in the relative severity of injury between the groups, but it is evident that early surgery was not harmful in this trial. Thus, surgical decompression should be considered the first significant neuroprotective goal of SCI therapy in concert with other guidelines and potential experimental treatments.
20.2.2 Support of Blood Pressure
Ischemia is a prominent pathomechanism of SCI secondary injury. Spinal cord blood flow (SCBF) is normally autoregulated so that flow matches metabolic demand over a range of blood pressures (BP). However, after SCI, autoregulation is disrupted, and SCBF becomes more linearly linked to systemic BP [324]. SCI patients frequently have low BP due to neurogenic shock. Inadequate BP is a risk factor for poor neurologic patient outcome [150, 205, 330], presumably by exacerbating ischemic damage. Therefore, support of adequate BP is a key to effective neuroprotection. Recently, it has been shown that intraspinal tissue pressure may be used to guide the mean arterial pressure to optimize spinal cord blood flow [341]. This type of monitoring is well established in brain injury and it is logical to extrapolate to SCI [333].
20.2.3 Infection
Recent studies have provided evidence that SCI patients with infections such as pneumonia have less neurological recovery [95]. Thus, infection is a “disease-modifying factor.” Schwab and colleagues developed rodent models where an acute immune deficiency state associated with SCI increased vulnerability to infection due to alterations in monocyte function. This has been described as the spinal cord injury-induced immune depression syndrome (SCI-IDS) [42, 265, 266]. Additionally the risk of bacterial infection seemed to be injury-level dependent in an SCI mouse model [42]. A current prospective, multicenter, international clinical protocol, the SCIentinel study, is examining the hypothesis that the severity of immune depression is dependent on injury severity and injury level [180]. Based on this evidence, avoidance of infection is critical in SCI and could be neuroprotective. Recently, it has been learned that the population of bacteria in the gut is altered by SCI [136]. Emerging studies are assessing the impact of the altered microbiome on neurological recovery and complications.
20.2.4 CSF Drainage
CSF drainage is known to influence SCBF and has achieved prominence as a preemptive strategy to reduce paralysis due to spinal cord ischemia during aortic surgery. The ability of CSF drainage to improve SCBF after SCI is less certain. Spinal cord perfusion pressure (SCPP) is calculated to be proportional to the average pressure head (mean arterial blood pressure – MAP) minus the venous resistance that has been estimated as cerebrospinal fluid pressure (CSFP). Thus, by reducing CSFP, SCBF may be increased. In clinical studies of thoracoabdominal aneurysm repair combined with CSF drainage, an 80 % reduction in the relative risk of postoperative neurological deficits has been reported [63, 65, 316]. After SCI, efforts to maintain SCBF include correction of spinal shock due to lack of sympathetic tone and increased capacitance by keeping MAP >90 mmHg using vasopressors. Pharmacologically increased MAP is thought to compensate for the failure of SCBF autoregulation due to loss of tonic activity of sympathetic neurons. Lowering CSFP may be another alternative to increasing SCBF. A prospective randomized study including 22 patients evaluated the safety and feasibility of CSF drainage and the correlation with neurological recovery at 6 months post-SCI [186]. A CSF drain was placed prior to surgical decompression and maintained for 72 h in all subjects. Subjects were randomized to have CSF drained or not drained, with a drainage threshold of 10 mmHg (the typical value of CSF pressure). After rostral decompressive surgery, there was a marked increase in distal CSFP as well as the onset of a pulsatile waveform. These changes indicated that the CSF measurements prior to surgical decompression (recorded from the lumbar cistern) did not represent the entire CSF space due to rostral occlusion by unreduced bone and disc fragments. Thus, calculations of SCPP prior to decompression were inaccurate, and a reduction in apparent SCBF occurred after decompression when more accurate CSFP values were recorded. In this study, the CSF drainage was conservative; the CSFP remained well above the target value due to restrictions on the rate of drainage per hour and drain closure when intensive patient assessment by nursing was unavailable. Although the study was not powered to assess efficacy nor the patients stratified according to injury level, the authors conclude that there was no neurological detriment or benefit due to the intervention. In a recent study, a pressure recording catheter was inserted into the intrathecal space and then “wedged” between the damaged spinal cord and the dura. The pressure reading was much higher than that of the CSF and considered to represent elevated spinal cord tissue pressure [341]. This finding indicates the weakness of relying on oversimplified formulae for SCPP that assumes spinal cord tissue pressures are reflected by CSFP. A recent porcine SCI study has shown that neither elevating MAP with pressors nor releasing CSF alone improved SCBF other than transiently, but the combination of both manipulations increases SCBF in a more sustained manner [228].
20.2.5 Therapeutic Hypothermia
The impact of tissue cooling on ischemia in the brain and spinal cord has been studied for more than six decades. Interest in hypothermia partially derived from nature because temperature and metabolic rates are linked. Hibernating mammals dramatically reduce their core body temperature minimizing their metabolic needs. A key idea is that by reducing metabolic requirements, tissue survival during states of low blood flow is improved. Studies in anesthetized dogs by Bigelow and colleagues showed a nearly linear relationship between core temperature and reduced oxygen consumption down to the lowest survivable temperatures (28°C) [29]. Pontius, DeBakey, and colleagues reported that in dogs cooled systemically to 29.4°C, the incidence of post-thoracic aorta occlusion-related paralysis was reduced from 65 % to zero [253]. In the 1960s, hypothermia was employed for neuroprotection to allow temporary circulatory arrest for complicated aneurysm surgery [81]. An important advance was the finding that that mild hypothermia (e.g., 33°C), which is much safer clinically, also had significant neuroprotective effects in rodent stroke models [44]. As of 2016, hypothermia has been studied extensively in several clinical settings. The most compelling current clinical data to support a neuroprotective benefit for hypothermia are from clinical trials in post-cardiac arrest comatose patients [27]. Hypothermia has been tested extensively in traumatic brain injury (TBI) and to a lesser extent in SCI. Despite experimental evidence of reduced tissue loss and improved brain function in animal models [75], multiple clinical trials have failed to show a benefit of post-traumatic hypothermia in adult or pediatric brain trauma [271], other than for transient control of raised intracranial pressure. SCI studies commenced in the 1960s. Maurice Albin and Robert White created one of the first neurocritical care units, located at the Cleveland Metropolitan General Hospital. Numerous experiments examining the impact of hypothermia on the brain and spinal cord were performed. In a study published in 1968 [5], primates were subjected to weight-drop contusion SCI, and after a delay of 4 h, one group underwent local perfusion cooling with a target spinal cord temperature of 10°C maintained for 3 h. The results were impressive, all treated animals recovered, and none of the controls showed substantial recovery. Now, with decades of additional experience, the 1968 results with localized hypothermia seem too good to be true, in part due to increased skepticism about extremely distinct results. In other animal models, hypothermia has been shown to mitigate several aspects of secondary injury cascades. There is modulation of inflammation [76], with reduced neutrophil infiltration [52], less glutamate release [166] and excitotoxicity, and reduced cellular energy metabolism [352]. The preponderance of experimental evidence indicates systemic cooling methods to be more effective [212] than epidural cooling [48].
Several small clinical studies of spinal cooling have been performed, but none has had a sufficiently robust design to provide conclusive evidence of efficacy due to small patient cohort numbers and a lack of suitable controls. The use of hypothermia is greatly underreported based on discussions with senior neurosurgeons who participated in unpublished studies. Since it is not a drug, it is relatively easy to apply hypothermia. Clinical experiments in the 1970s emphasized locally applied cooling due to the influence of Albin and White’s publications. Bricolo and colleagues treated eight neurologically complete acute SCI patients with 2 h of subdural cooling using 5°C saline irrigation. Motor and sensory recovery was reported in four subjects with 2 recovering ambulation [41]. Hansebout and colleagues studied the effect of local epidural cooling in a dog model of spinal cord compression to assess optimal treatment duration [182, 340]. Based on favorable results with 4 h of cooling, they then recruited ten patients with complete SCI to undergo early surgical decompression and local extradural cooling within 8 h of complete SCI. The dural temperature at the injury site was maintained at 6°C for 4 h using a suspended extradural saddle (cord temperature was not recorded). A factor complicating interpretation was the delivery of dexamethasone, 6 mg every 6 h for 11 days, and then tapered until the 18th day. This study had a mean follow-up of 1.9 years. Of six subjects with thoracic SCI, four were unchanged, one became ambulatory, and another had sensory recovery. Of four subjects with a cervical injury, three were unchanged, and one showed motor and sensory recovery [148]. These studies occurred before the publication of the important ASIA impairment scale (AIS) in 1982. Hansebout and Hansebout published a long-term follow-up of the original cohort with ten additional patients enrolled in local cooling and dexamethasone treatment. Results were reported by adapting their previous scoring system to the AIS scale. From the total of twenty treated patients categorized as baseline AIS-A, there was improvement in thoracic injuries of 16.7 % to AIS-B, 33.3 % to AIS-C, 16.7 % to AIS-D, and a mean sensory descent of 2.5 levels per patient. For the cervical cohort, there was improvement of 35.7 % to AIS-B, 21.4 % to AIS-C, and 7.2 % to AIS-D, with a mean motor descent of 0.92 levels per patient [147]. These recovery rates are much better than the published natural history for cervical and thoracic injuries [302, 355], but we know that the reproducibility of small cohorts is poor and robust trial design is needed to obtain valid conclusions regarding therapeutic efficacy [194]. Critique of this study included the contradictory evidence available for the use of steroids, the possibility of improved outcomes as the natural evolution following early decompression surgery, and the interpolation of the clinical data [321]. Given the current information, it would be better not to combine steroids and hypothermia in a clinical trial.
Currently, systemic hypothermia after acute SCI in subjects with motor complete paralysis is under evaluation in an observational prospective cohort study sponsored by the University of Miami/The Miami Project to Cure Paralysis (ClinicalTrials.gov identifier: NCT01739010) at Jackson Memorial Hospital, Miami, FL. Systemic cooling is achieved using an intravascular cooling catheter advanced centrally through the femoral vein. The target temperature is 33°C at a maximum cooling rate of 0.5°C/h, MAP is maintained >90 mmHg, and rewarming begins after 48 h at a rate of 0.1°C/h. A preliminary report of 14 patients scored as AIS-A on admission indicated conversions of three patients to AIS-B, two to AIS-C, and one to AIS-D, with similar rate of complications to the historical control group [204]. In a more recent publication [74], data from 35 subjects with initial AIS-A cervical injury was presented. The rate of conversion to higher AIS grades exceeds than expected from historical controls. Both studies indicate a delay to initiate cooling with an average of 5.8 h for subjects rapidly transferred to the institution, followed by 2.7 h to achieve the target temperature. All patients underwent surgical decompression at an average of 16.5 h after injury. There was no relationship between the timing of cooling onset and neurological outcome. The majority of complications were pulmonary, and the incidence was similar to that in recent series if adjusted for injury severity. Although the data is promising, it remains difficult to isolate the efficacy of hypothermia without a randomized control group. A new study, acute rapid cooling of the traumatically injured cord (ARCTIC), is designed to randomize patients to control and cooling temperature groups.
20.2.6 Dietary Adjuvants/Restrictions
There has been interest in the influence of dietary factors on CNS inflammation and oxidative stress and diet’s contribution to neurodegenerative diseases such as Alzheimer’s [145, 221] and epilepsy [173]. The positive influence of a ketogenic diet on the incidence of seizures in children has been known for a hundred years [153] and the favorable impact of fasting for millennia. Ketogenic diets reduce circulating glucose and increase ketones as an energy substrate in the CNS. The ability of a ketogenic diet to improve cerebral metabolism after TBI was reviewed by Prins and Matsumoto [260]. In rodents, ketones can reduce free radical generation from mitochondria during stress induced by excess levels of glutamate [218]. Reduction in reactive oxygen species (ROS) has also been observed with caloric restriction. Following unilateral cervical crush hemisection, every-other-day fasting (EODF) leads to reduced injury size, increased gray matter sparing, improved ipsilateral forelimb function, increased functional Tropomyosin receptor kinase B (trkB) receptor levels, and increased plasticity of corticospinal tract (CST) axons [252]. EODF animals weighed 8 % less than controls at the end of the experiment. Blood glucose and β-hydroxybutyrate ketone levels varied inversely throughout the study.
In a rat model of cervical hemi-contusion, Tetzlaff and colleagues mimicked the effects of fasting using a 3:1 ratio regimen of ketogenic diet starting 4 h after injury, with a return to standard diet 12 weeks post-injury. Animals receiving this diet showed improved behavioral recovery, reduced lesion size, increased gray matter sparing, with an increase in the vascular transporters GLUT1 and MCT1 [310]. The post-injury weight gain was similar between the ketogenic and control group. These investigators also studied daily food restriction to 75 %, equivalent to the total food intake of EODF animals, after T10 moderate contusion injury. This continuous caloric restriction did not yield evidence of protection, whereas EODF improved locomotor recovery [168]. When EODF was studied in a mouse model, no behavioral or histological improvement was observed after a T10 crush SCI, and β-hydroxybutyrate levels failed to rise substantially in the first week post-injury despite prominent loss of body weight [311]. These data indicate that the responses in mouse and rat models differ; thus, caution should be exercised in the extrapolation of rodent data to humans.
Creatine is a popular health supplement whose delivery has been shown to maintain ATP homeostasis in TBI models. Rabchevsky and colleagues tested the neuroprotective effect of 2 % creatine supplementation of rat chow given for 4–5 weeks prior to T10 New York University (NYU) or Infinite Horizon device-delivered (IH) contusion injuries on tissue sparing and locomotor recovery (see chapter 25). They also tested the effect of continuation or cessation of the diet after the T10 IH injury. Although no overall effects on locomotor recovery or white matter sparing were found, the creatine supplemented groups in the IH experiment had smaller lesion volumes and more gray matter sparing [261]. IH injuries were found to be more focal in distribution than NYU injuries. These differences provide another example of the variations arising in “standard” injury models and the caution that is needed in extrapolating results for human trial design where injuries are heterogeneous [85].
The neuroprotective effects of long-chain omega-3 polyunsaturated fatty acids (PUFAs) such as docosahexaenoic acid (DHA) are being studied. The effects of acute IV delivery of DHA at 30 min, 1 h, and 3 h post-injury were investigated in a T10 spinal cord compression model. Thirty-minute but not 3 h delivery was associated with substantial neuroprotection with improved neuron and oligodendrocyte survival, reduced inflammation, and significantly improved locomotor scores. Reductions in the oxidation of lipids, proteins, RNA and DNA, and COX-2 expression were found [159], as well as evidence of reduced axonal cytoskeletal damage, demyelination, and preservation of serotonergic innervation below the injury level [338]. A recent study performed in a T6/7 mouse clip compression model found reduced edema, tumor necrosis factor (TNF)-α, nitrotyrosine formation, and glial fibrillary acidic protein (GFAP), FAS ligand, BCL2-associated X protein (BAX), and B-cell lymphoma 2 (BCL2) expression when DHA was administered 30 min after injury [245]. Translation of this therapy has been limited due to lack of cGMP (current good manufacturing practice) grade DHA indicating the difficulty of obtaining clinical grade formulations that affects many agents suitable for clinical testing. The therapeutic window for DHA is brief, apparently true of most neuroprotectants, and its strong safety profile supports acute administration.
20.3 Pharmacological Strategies Tested in Human Clinical Trials
20.3.1 Methylprednisolone Sodium Succinate (MPSS)
Studies with MPSS have been important in the evolution of SCI clinical trials raising critical questions about the benefit/risk equation for therapeutic neuroprotection, the relative weight to be assigned to robust prospective research designs as compared to other forms of cohort studies [102] and the importance of a substantial body of evidence in justifying an emerging treatment as a treatment standard. The student of neuroprotection is referred both to the many reviews of this topic and also to the primary studies and subsequent critiques [160], many of high quality. As an anti-inflammatory drug, there are numerous indications for methylprednisolone and a long history of clinical use in the CNS [234]. Likewise, complications related to its use have been reported for almost 40 years [312]. After experimental SCI, MPSS administration was shown to inhibit lipid peroxidation [142, 143], facilitate extracellular calcium recovery [348], and reduce inflammatory responses [158], white matter neurofilament degradation [40], and macrophage/microglia infiltration [239]. Given the large data set indicating beneficial effects in preclinical studies and the absence of an effective clinical treatment for acute SCI, there was enthusiasm for clinical translation of MPSS. There were three prospective randomized studies of MPSS in SCI lead by Bracken and colleagues. The First National Acute Spinal Cord Injury Study (NASCIS I) was initiated in 1979 and completed in 1984. No significant difference in improvement was found between subjects that received doses of 1000 mg or 100 mg of MPSS for 11 days [35, 38]. However, the high dose was associated with an increased relative risk for case fatality and wound infection. The NASCIS II study increased the dose and reported neurological improvement for MPSS versus placebo if the treatment was started within 8 h of injury (30 mg/kg loading dose, 5.4 mg/kg/h for infusion continued for 23 h) [36, 37]. A third NASCIS trial was published in 1997, in which a 24-h treatment period was compared to a 48-h treatment course. There was no placebo control, and MPSS was compared to trilizad, a non-glucocorticoid lipid peroxidation inhibitor. The trial included the Functional Independence Measure as well as ASIA motor and sensory scores as principle outcomes. A Japanese multicenter trial reported following NASCIS II, using the same pharmaceutical protocol, also found a benefit in the MPSS group [238]
A decade after publication of the NASCIS II data, the use of MPSS was widespread and in some cases not supported by the clinical trial evidence. A counter opinion framed MPSS as an inappropriate “gold standard” treatment in acute SCI care. Viewed retrospectively, this early assignment of “gold standard” status is considered to have been unwise and damaging to the study of other therapeutics [160]. However, at the time the excitement that something worked in SCI overshadowed a more measured evaluation of the study results. NASCIS III indicated an advantage to prolonged (48 versus 24 h) treatment if drug delivery was started 3–8 h post-injury, but no advantage if initiated within 3 h [39]. When the NASCIS II protocol was tested in France, no significant differences in outcomes between methylprednisolone and nimodipine were found [247]. A small prospective trial in Japan assessed complication rates with the NASCIS II MPSS protocol versus placebo in patients with cervical injury (and associated cervical stenosis) that did not undergo surgical decompression. A higher rate of pneumonia and GI bleeding was observed in the MPSS group. It should be noted that the average patient age was 61 years old [230]. Further, seven retrospective studies took place in the 1990s, six in the USA and one in Ireland; these have included more than 1300 patients and have shown equivalent motor outcomes in between the steroid and non-steroid groups with slight trends or significance toward the increase of complications in the MPSS groups [119, 121, 122, 152, 206, 257, 259].
MPSS is the most extensively tested pharmacological neuroprotective agent in the SCI field, but evidence to support its use in acute SCI for either the 24- or the 48-h regimen remains controversial due to issues in the research designs and the incidence of associated complications. Three multicenter, randomized clinical trials were completed to address the efficacy of MPSS, and only the NASCIS II has clearly indicated a benefit for neurological scores. The potential increase in serious medical complications including infections, sepsis, respiratory complications, pulmonary embolism, gastrointestinal hemorrhage, pancreatitis, delayed wound healing, worsening of head injury outcomes, and death is a concern. Furthermore, access to the study data has been limited, and concerns have been raised about the control group results and the lack of submission of the data to the FDA to obtain an approved indication [60]. Altogether, these critiques seem harsh treatment of large well-intentioned RCTs, but given the high incidence of serious complications in SCI [133], it is unwise to use an agent that may add to this morbidity. In summary, due to the limited evidence of efficacy in clinical trials and the risk of medical complications, the use of MPSS is only recommended by the American Association of Neurological Surgeons/Congress of Neurological Surgeons (AANS/CNS) as an option which may be supported by clinicians balancing the evidence of side effects toward the limited clinical evidence and the circumstance that there might be no other available therapeutic to offer at the moment [336].
20.3.2 GM-1 (Monosialotetrahexosylganglioside)
GM-1 belongs to a family of glycosphingolipids that contain only one sialic acid residue; gangliosides are abundant in the nervous system [73, 146]. GM-1 is believed to have trophic effects. These include inducing neurotrophin-3 (NT3) release with further activation of the TrKC receptor [262], mimicking nerve growth factor (NGF) activity, and activation of the TrKB receptor [104]. Synthetic derivatives of GM-1 – LIGA4, LIGA20, and PKS3 – have been shown to protect neurons from glutamate-induced death [223]. Clinically, GM-1 was tested in stroke before SCI [9]. Despite several trials in stroke, its efficacy remained unclear [SASS trial] [113], possibly due to methodological issues [7], not unlike those that affect SCI clinical trials.
The first clinical report testing GM-1 in SCI was a small prospective, randomized, placebo-controlled, double-blinded trial [117, 118]. Thirty-seven patients received a loading dose of MPSS 250 mg followed by 125 mg every 6 h for 72 h, together with GM-1 100 mg/day within 72 h post-SCI, continued daily for 18–32 days. Thirty-four patients were available at 1-year follow-up, and the authors reported a significant improvement in AIS motor scores (p = 0.047) and Frankel grade (p = 0.034) with no adverse effects attributed to GM-1. The following year, the Sygen multicenter, prospective, double-blind, randomized, and stratified study was initiated. The Sygen study is one of the most important trials the SCI field has performed and the largest SCI therapeutics trial ever conducted. 797 patients were enrolled from 1992 to 1997. All patients were first treated according to the MPSS NASCIS II protocol and then randomized to placebo, low-dose GM-1 (300 mg loading dose and then 100 mg/day), or high-dose GM-1(600 mg loading dose and then 200 mg/day) for 56 days [116]. Follow-up with AIS and Benzel classification continued until 52 weeks post-injury. The primary endpoint of the study was “marked improvement” at 26 weeks, defined as a two-grade change in the Modified Benzel Classification from the baseline AIS grade. Although there appeared to be a more rapid neurological recovery in the Sygen-treated group, there was no difference in the overall treatment groups at 26 weeks. However, several interesting trends were observed in the data including more improvement in the AIS-B treatment group and efficacy trends in sensory, motor, bowel, and bladder function as well as ceiling effects in subjects that were initially AIS-C and AIS-D. A ceiling effect means that, e.g., a subject enrolled as AIS-D cannot show a 2 grade improvement and should not have been recruited to the trial. Adverse events did not differ between the treatment groups. There was no true placebo in the GM-1 study because MPSS was considered the gold standard at that time. In summary, the pivotal clinical trial for GM-1 failed to meet its prospectively set primary outcomes. The primary efficacy measure of the Sygen study was a high bar, and statistically significant differences were only observed on post hoc analysis. There is no subsequent clinical study available to validate or disprove the previously mentioned clinical evidence nor has much further experimental evaluation been published [47]. Given the logistical investment to conduct a phase 3 RCT in SCI, it is rare that a therapeutic is further tested after the failure of a well-designed study, even if redesign or stratification might lead to a different result. Currently a GM-1 loading dose of 300 mg followed by 100 mg/day for 56 days after the administration of methylprednisolone within the first 8 h of injury (as per NASCIS II protocol) is recommended by the AANS/CNS as an option (if available) for the treatment of acute SCI. Data from the GM-1 study has proven useful as a comparator for other clinical studies and to help define the natural history of SCI recovery [97, 302]. One of the most striking aspects of the GM-1 story is the paucity of published preclinical data in SCI models prior to the initiation of clinical trials. In the only study reporting the combination of MPSS (30 mg/kg) and GM-1 in a rat model of contusions of graded severity, the acute combination of both drugs blocked the neuroprotective effects of MPSS. In that study, no effect of acute administration of GM-1 was noted, and MPSS alone was neuroprotective in mild and moderate contusion but had minimal benefit after severe contusion [61].
20.3.3 Magnesium
Magnesium salts and polyethylene glycol are used extensively in medicine. Magnesium neuroprotective properties have been evaluated in animal models for 25 years [167]. Magnesium can block N-Methyl-D-aspartate (NMDA)-type glutamate receptors and reduce calcium influx to cells attenuating glutamate excitotoxicity [361]. Other reported effects include decreased apoptosis [200], improved electrophysiological conduction, reduced lipid peroxidation [315], and normalized lactate levels after SCI [240].
After brain and spinal cord injury, local levels of magnesium are depleted contributing to mitochondrial dysfunction. In rodents intraperitoneal high doses of MgSO4 (600 mg/kg) given immediately after SCI have resulted in early functional recovery and preservation of spinal cord ultrastructure when compared to small doses (100 mg/kg) [174]. Although the effects of magnesium are noteworthy, the doses postulated to induce neuroprotection may be too high and could produce respiratory depression or cardiac arrest in the acute clinical injury setting. Additional preclinical studies assessed magnesium formulations with more efficient blood-brain barrier penetration. One strategy is to combine magnesium salts with polyethylene glycol (PEG) to increase penetration. PEG can have several effects on drug delivery, including increasing the serum half-life of nanoparticles, enhancing brain barrier permeation, and directly supporting membrane repair acute SCI [32]. Several patents have described pharmacological properties of Mg-PEG compounds that achieve higher CSF concentrations as compared to Mg alone, optimal IV delivery rates, and calculations of Cmax, AUC0-∞, and T1/2 for different PEG-magnesium formulations (US Patents 8840933, 8852566). A series of animal studies has examined the relative effects of PEG, Mg2+, and their combination compared to saline after SCI on tissue preservation and neurological outcomes. Kwon and colleagues conducted a detailed study to assess the neuroprotective effects of either MgCl2 or MgSO4 combined with PEG at two different doses, 127 μmol/kg or 254 μmol/kg across four time points for initial delivery: 15 min, 2, 4, and 8 h after injury. Two, four, or six infusions spaced 6 or 8 h apart were compared. Using lesion size as a marker of neuroprotection, it was found that early delivery was more protective, the anion (Cl versus SO4) made no difference, the higher concentration was superior, more IV doses were superior to fewer, and a 6-h dose infusion interval was best [186, 188]. Other groups have reported improved tissue sparing with MgSO4 (300 mg/kg) + PEG versus saline controls in a severe T3 clip compression injury, with no significant differences when compared with either treatment alone [80].
Recognizing that IV CNS magnesium therapy could be limited by low CSF and tissue levels, and the previously reported results by Kwon and colleagues, Medtronic Inc. developed a polymer formulation that enhances magnesium accumulation at the injury site. Acorda Therapeutics licensed this formulation as AC105 (Mg/PEG) and designed a phase II double-blinded, randomized, placebo-controlled study of the safety, tolerability, and potential therapeutic activity of a regimen of six sequential AC105 doses in patients with acute SCI. The first dose was to be given in progressively earlier cohorts, 12, 9, and 6 h after injury with all doses delivered over 30 h (ClinicalTrials.gov Identifier: NCT01750684). Although the study was terminated in 2015, no results have been yet disclosed. Multiple other studies of magnesium in various indications have reported mixed results. A recent large-scale trial (1700 subjects) assessing the prehospital use of magnesium sulfate in stroke did not find a therapeutic effect [274].
20.3.4 Riluzole
Riluzole is an extensively studied off-patent benzothiazole drug. The neuroprotective effects of riluzole were first reported in ischemia [222]. It counteracts glutamate-mediated neurotoxicity by inhibiting the release of glutamate from presynaptic terminals [56, 227], increasing high affinity glutamate uptake [11], and inhibiting sodium channels, by maintaining voltage-regulated sodium channels in the inactivated state [23, 258]. Riluzole crosses the blood-brain barrier [22]. The molecule was developed in France as an antiepileptic, and there have been several clinical trials for psychiatric conditions such as generalized anxiety disorder, major depressive disorder, and obsessive-compulsive disorder [128]. The only FDA-approved indication of riluzole is to prolong the time interval before assisted ventilation is needed in amyotrophic lateral sclerosis (ALS) patients [233]. Given the established safety record and known safe dose levels, the regulatory pathway to test such drugs in SCI can be much simpler than for novel therapeutics.
Experimental studies have assessed the effect of riluzole delivery in a variety of SCI models. Excessive glutamate is neurotoxic by leading to accumulation of intracellular Ca2+ that activates calpain proteases that break down cytoskeletal components such as spectrin, Microtubule-associated protein 2 (MAP-2), and neurofilament. Pretreatment of rats with 8 mg/kg of riluzole 15 min before and 2 h post 25 g/cm NYU impact to the T10 spinal cord substantially reduced activated calpain and MAP-2 loss in neurons [297]. Four or 8 mg/kg before and at the onset of reperfusion in aortic cross-clamping prevented ischemia-induced necrosis and cytoskeletal proteolysis [195]. Rats receiving riluzole (2 mg/kg 30 min post-thoracic compression and then BID for 10 days) recovered somatosensory evoked potentials (SSEPs) and had smaller lesion volumes [313]; 5 mg/kg 15 min after compressive SCI improved gray matter and selective white matter sparing with better hind-limb coordination and strength [278]; 8 mg/kg post S2 transection reduced spasticity [177], but 10 mg/kg caused motor weakness. Only two studies assessed a clinically meaningful therapeutic window, 8 + 30 mg/kg of MPSS, 2 and 4 h post-contusion promoted tissue sparing and recovery in Basso, Beattie, and Bresnahan (BBB) scores [235]. Eight mg/kg BID 1 and 3-h post-C7 cervical clip compression and continued at 6mg/kg BID for 7 days showed improved SSEPs, tissue preservation, reduced apoptosis, and improved locomotor scores [344]. In this study, a striking difference in the benefit between the 1- and 3-h post-injury groups is observed indicating the brief therapeutic window to reduce secondary injury.
In 2010 the North American Clinical Trials Network (NACTN) initiated a prospective multicenter phase I trial to evaluate the pharmacokinetics, safety, and effects of riluzole in acute SCI (ClinicalTrials.gov Identifier: NCT00876889). Thirty-six patients were enrolled; the dose was 50 mg PO within 12 h of injury, continuing BID for 14 additional days. The trial did not have a concurrent control group, but the comparison group was formed by 36 matched patients meeting the eligibility criteria that received standard of care treatment in NACTN centers from October 2005 to November 2012 enrolled to the NACTN data registry. The authors report a significantly higher mean motor score in cervical patients treated with riluzole (p = 0.021, 31.2 points versus 15.7 points in the control group) from admission to 90 days, with no serious adverse events or deaths related to riluzole [132]. A phase 3 study is now underway for riluzole. A well-designed multicenter, double-blind, randomized, placebo-controlled trial will test a dose of 100 mg BID during the first 24 h post-SCI, followed by 50 mg BID (day 2–14 post-SCI) in an international study enrolling subjects with acute cervical SCI [99] (Clinical trials.gov identifier: NCT01597518). The study is aimed to be completed in 2018.
20.3.5 Minocycline
This broad-spectrum bacteriostatic antibiotic is the most lipid-soluble tetracycline available and has excellent penetration into the CNS. Minocycline has gained interest for its neuroprotective and anti-inflammatory properties in several neurodegenerative diseases including Huntington’s [54], Parkinson’s [163, 164], schizophrenia [53], and multiple sclerosis [360]. In animal models of SCI, minocycline has been shown to reduce caspase-3 activation, attenuate cell death, reduce lesion size [202], decrease activated microglia/macrophage density, oligodendrocyte death and CST dieback [306], inhibit cytochrome-C release and astrogliosis [326], and inhibit lipid peroxidation [295]. Minocycline moved rapidly through preclinical testing to clinical evaluation due to its prior extensive safety record as an antibiotic and established toxicity limits.
From 2004 to 2008, the University of Calgary conducted a single-center, double-blind, randomized, placebo-controlled, phase II study, to test the safety and estimate outcome changes after an IV minocycline infusion started within 12 h of SCI. Surgical decompression occurred during the first 24 h post-SCI, and minocycline was delivered IV BID until 7 days post-SCI (ClinicalTrials.gov identifier: NCT00559494). MPPS was not given to the subjects. Each had a lumbar catheter placed to allow CSF sampling. To achieve serum levels similar to those found to be effective in experimental models, the authors treated the first five subjects with an IV loading dose of 200 mg, followed by 200 mg BID for 7 days, and then assessed serum and CSF minocycline trough and peak levels. Based on this information, the dose was then revised to an 800 mg loading dose, reduced by 100 mg in each subsequent dose until 400 mg, and then maintained BID for the remainder of the 7 days. The authors found no statistically significant difference in the primary outcome (ISNCSCI motor scores up to 1 year) [49]. Only one subject showed a minocycline linked adverse event with a transient elevation of liver enzymes. Although the study was not powered sufficiently to prove therapeutic efficacy, patients with complete cervical SCI showed a 14-point motor increase and patients with incomplete cervical SCI a 22-point improvement over placebo. Post hoc analysis indicated that the higher minocycline doses were associated with a greater degree of motor recovery. These data shows the importance of obtaining pharmacokinetic data from CSF to assess the adequacy of dosing strategies. A related serum biomarker study showed that subjects receiving minocycline had lower levels of the light chain of neurofilament than those receiving placebo [184]. Minocycline is now being assessed in a multicenter, randomized, double-blind, placebo-controlled Canadian phase 3 trial enrolling subjects with acute cervical SCI. Minocycline will be infused centrally BID at 800 and 700 mg on day 1, 600 and 500 mg on day 2, and 400 mg thereafter from day 3 through 7 (Clinical trials.gov identifier: NCT01828203). An estimated enrollment of 248 subjects is aimed to be completed in 2018.
20.3.6 Cethrin
RhoA is a major G-protein regulator of the cytoskeleton. Rho’s activity state is regulated by whether it’s in the GTP (active) or GDP (inactive) state. Active RhoA promotes growth cone collapse by activating Rho kinase and also activates apoptosis in the injured spinal cord [83]. C3 transferase, a bacterial enzyme, ADP ribosylates asparagine 41 in the effector-binding domain of Rho, irreversibly preventing its activation [72], one result of which is to prevent axonal growth cone collapse when confronted by inhibitory molecules in the spinal cord. Cethrin is a synthetic molecule that consists of C3 transferase with an added cell membrane transport sequence [214, 343]. Key experiments included in vitro studies where primary retinal neurons treated with C3 transferase extended neurites on myelin substrates. After optic nerve crush and C3 transferase treatment, axons crossed the lesion and grew distally [203]. Experiments in a mouse hemisection model of SCI with C3 transferase showed significant differences in the modified BBB analysis and long-distance regeneration of CST fibers [72]. The cell membrane transport sequence enhanced dural penetration in rodent studies. This sequence alteration is claimed to make extradural delivery through the dura feasible [214].
The Rho inactivator BA-210 was trademarked as Cethrin by BioAxone BioSciences Inc., and a phase I/IIa multicenter, nonrandomized, open-label, dose-escalation trial for patients with complete cervical or thoracic SCI (ClinicalTrials.gov Identifier: NCT00500812) was conducted [100]. From the 48 patients enrolled, 32 completed the 12-month follow-up. Treatment was delivered during surgical decompression with Cethrin mixed within a fibrin sealant placed on top of the dura mater. Five doses were tested, 0.3, 1.0, 3.0, 6.0, and 9.0 mg. Most of the subjects were treated between 24 and 72 h after injury [231]. The most compelling data from the study is a dose-dependent effect on recovery of cervical motor scores with the maximum observed recovery with the 3 mg dose (27 points improved from baseline). Anderson and McKerracher analyzed the data from pooled dose groups using the method of last observation carried forward and compared to the Sygen control group, the European Multicenter Study about Spinal Cord Injury (EMSCI) database, the US Models Systems and Waters published recovery data. The analysis showed a conversion rate from AIS-A to AIS-C of 33 % in cervical patients and 7 % in thoracic patients, the cervical conversion rate being higher than reported in the Sygen control group. The cervical total motor score improvement of 18.6 ± 17.3 exceeds the other data sets, although the EMSCI recovery rates are close. More than 40 % of cervical subjects in the pooled Cethrin group recovered 2 or more neurological levels, which is also higher than usual. BioAxone announced a multicenter, randomized, double-blind, placebo-controlled phase IIb/III trial for Cethrin to begin for patients with acute cervical SCI (ClinicalTrials.gov Identifier: NCT02053883), aimed for completion in 2016. Subsequently, BioAxone licensed Cethrin to Vertex Pharmaceuticals Incorporated in late 2014 and withdrew the study. Cethrin appears now under the name of VX-210, and a new phase IIb/III study (randomized, double-blind, placebo-controlled) sponsored by Vertex launched in early 2016 for patients with acute cervical SCI. The trial is expected to be completed in 2018 (ClinicalTrials.gov Identifier: NCT02669849).
20.3.7 Naloxone
In the late 1970s, the opiate antagonist naloxone was found to reverse the septic shock-like consequences of IV delivery of endotoxin. B-Endorphin, an opiate agonist, was released in response to shock [155]. In 1981, Faden, Jacobs, and Holaday published an experiment in cats where, following a severe C7 cervical contusion, naloxone or saline was delivered 45 min after injury. Animals receiving naloxone recovered blood pressure rapidly and showed improved forelimb and hindlimb function 3 weeks after the injury. The authors inferred that improved SCBF was the therapeutic mechanism [91]. Further animal studies showed a reduction in ischemia, preserving SCBF and SSEPs [108, 349]. Naloxone also reduced superoxide formation by reactive microglia and antagonized dynorphin A, an endogenous opioid capable of inducing hindlimb paralysis [90], and reestablished normal extracellular calcium levels after SCI in dogs [308]. However, other researchers were unable to verify naloxone mediated improvements on SCBF in rodents with clip compression injury [335]. A small human dose-escalation trial recruited 29 patients and did not show statistical significance in recovery, but had a trend toward SSEP improvements and motor recovery in patients receiving high doses of naloxone (200 mg/m2 loading dose followed by 150 mg/m2/h for 23 h infusion) [107]. There were no serious adverse events except increased pain perception in subjects who had additional injuries such as limb fractures and who were neurologically incomplete. In 1990, naloxone was included as one of the NASCIS II trial arms (5.4 mg/kg loading bolus and 4 mg/kg/h infusion for 23 h) along with MPSS and placebo. No statistically significant evidence of improvement in motor or sensory function was detected [36, 37]. As with many other potential neuroprotectants, it’s hard to predict the therapeutic window in humans, when the effective window in animals is brief, e.g. 30–60 min. There is a tendency to extrapolate that the window will be longer in humans, but the arguments for this are not persuasive, and efforts to provide the earliest possible safe delivery are needed if neuroprotection is to be effective.
20.3.8 Gacyclidine
Gacyclidine (GK-11 Beaufour-Ipsen Pharmaceutical, Les Ulis, France) is a noncompetitive NMDA receptor antagonist with neuroprotective properties including prevention of glutamate-induced neuronal cell death [82] and stabilization of synaptic currents [332]. Efficacy in SCI animal models was shown in dose-response studies indicating increased functional recovery, reduction in cystic cavitation and astrogliosis [103], higher SSEP amplitudes [115], and an optimal therapeutic window when administered within 30 min of injury [154]. In 1999 a phase II randomized, double-blind, placebo-controlled clinical trial in France was completed; 280 patients were enrolled to receive escalating doses of 0.005, 0.01, and 0.02 mg/kg of GK-11 via IV infusion within 2 h of injury with a second dose within the next 4 h. The analysis showed a nonsignificant trend for a benefit at 30 days in incomplete subjects with cervical injury receiving 0.2 mg/kg. However, this was not evident over placebo at the 1-year follow-up. This study remarkably initiated a neuroprotective strategy within 2 h of injury [319, 320]. Further SCI studies with GK-11 have not apparently been pursued.
20.3.9 Thyrotropin-Releasing Hormone (TRH)
TRH, a tripeptide produced by the hypothalamus, was found to have anti-inflammatory, antioxidant, and membrane-stabilizing properties following SCI [236]. It also became of interest due to its action as an opiate antagonist [156]. Faden and colleagues first tested the effects of TRH compared to dexamethasone in a cat contusion model as an alternative to naloxone. TRH is a partial opiate antagonist and thus has less analgesic blocking properties than naloxone. Blockade of analgesia was a concern in the clinical use of naloxone in acute SCI. Higher scores in forelimb, hindlimb, and total functional outcomes were found in animals treated with TRH (p < 0.01) [92]. Other properties attributed to TRH from animal experiments include a dose-dependent reduction of leukotriene D4 [105], decreased intracellular pH, tissue cations and edema after trauma [94], increased CNS blood flow [181], excitotoxin antagonizing properties [263], and greater efficacy versus MPSS in tissue sparing [21]. In 1995, results of a small clinical trial for TRH were published by Pitts and colleagues; 20 patients were included in the trial and admitted within 12 h of injury to be randomized to receive TRH (0.2 mg/kg bolus continued by a 0.2 mg/kg/h infusion for additional 6 h) or placebo; both groups included complete and incomplete patients. The analysis took place using NASCIS and Sunnybrook scores at 1, 3, and 7 days and 1, 4, and 12 months post-SCI. The authors report higher motor (58.8 ± 11.9 versus 36.7 ± 23.5 placebo) and sensory (84.5 ± 4.1 versus 55.7 ± 22.7 placebo) scores in incomplete patients at the 4-month evaluation. Although the differences are statistically significant, the data was interpreted as not definitive due to the small sample size. Further, an important number of patients were lost for the 12-month follow-up, and data could not be considered to be informative for this timepoint. Additionally, there were no discernible effects in the complete SCI cohorts [251]. TRH as a neuroprotectant remains an open question.
20.3.10 Nimodipine
The dihydropyridine calcium channel blocker nimodipine has established clinical efficacy for the prevention of vasospasm after subarachnoid hemorrhage ([209, 249]). As raised intracellular calcium levels are damaging to cells affected by SCI [346], it is logical to test drugs that can block calcium channels. Following SCI in experimental animal models, it was found that administration of nimodipine lead to vascular dilation and reduction of blood pressure [140] unless combined with adrenaline [135] to increase adrenergic tone, in which case SCBF could be improved at the site of injury. Further experiments could not verify improvement in SCBF or electrophysiological conduction for either nimodipine or MPSS monotherapy [162, 268]; nimodipine was tested in a human clinical trial in France [247], involving 48 paraplegic and 58 tetraplegic patients randomized to receive: nimodipine (0.015 mg/kg/h for 2 h and 0.03 mg/kg/h infusion for 7 days), MPSS (as per NASCIS II protocol), both, or placebo. One hundred patients were available for the 1-year evaluation. Neurologic improvements were attributed to the natural evolution of the injury with no differences found in any group as compared to the placebo-treated group; the authors additionally reported no benefit from early decompression (8 h post-SCI) in a cohort of 49 patients.
20.4 Experimental Pharmacological Strategies
We now consider therapeutics that have considerable experimental data from animal studies but have not been tested clinically in SCI.
20.4.1 Erythropoietin
Erythropoietin (EPO) is a cytokine glycoprotein hormone produced by the kidney of adults that regulates red cell formation. EPO and its receptor (EPO-R) are expressed in neural tissues, especially neurons and glial cells [68, 77]. In the 1990s it was reported that EPO could reduce apoptosis in a stroke model [269] and that recombinant EPO improved endothelial survival and repair in the ischemic penumbra [208]. EPO and EPO-R are upregulated in the ischemic penumbra by a hypoxemia-inducible factor (HIF) [292]. After further preclinical testing, EPO was translated in clinical trials of ischemia due to stroke [86, 87] and vasospasm after subarachnoid hemorrhage [329]. Clinical efficacy in cerebral ischemia remains unclear [296], and concerns have been raised about the safety of coadministration of t-PA and EPO [356]. In a model of spinal cord ischemia, the immediate administration of EPO, during reperfusion, after 20 min of aortic occlusion, spared ventral motor neurons in a dose-dependent manner [50]. Injection of single doses of intraperitoneal EPO, 1-h post-contusion or clip SCI, was associated with motor recovery and reduced injury sizes [127]. In an NIH-sponsored independent replication study of these reported EPO effects, no differences in locomotor recovery were found [250], although there was a nonsignificant trend for epicenter tissue sparing. A difference in apoptosis was not assessed, but reported in the index study and other experimental studies of EPO, and could have provided a signal of biological effect. An additional study using the EPO derivative, darbepoetin, could not reproduce the therapeutic effects reported by Gorio et al. [225]. Numerous mechanisms of activity of EPO have been reported such as reduced inflammation by attenuation of IL-6 [4] release and attenuation of astrogliosis with reduction of phosphacan/RPTPζ/β [334]. Recent studies have shown that methylprednisolone prevents oligodendrocyte (but not neuronal) excitotoxin-mediated apoptotic cell death by causing glucocorticoid receptor and HIF-1-α/1-β to bind to the EPO promotor leading to its expression and upregulation of antiapoptotic Bcl-xL gene expression. This level of complexity indicates why it is easy for therapeutics trials to fail without robust iterative preclinical support as well as biomarkers of therapeutics activity. Failures to replicate preclinical studies do not necessarily mean that the therapeutic is ineffective but do suggest that the efficacy is lower than the index study. An endogenously active molecule such as EPO can be predicted to have multiple actions. Recently, two small clinical studies have been reported in which EPO was compared to MPSS. A modest superiority of EPO was reported [6, 64]. It is important that in larger studies EPO will be compared to placebo as there is increasing information that MPSS may worsen the transient immune deficiency associated with SCI [180].
20.4.2 Anti-CD11d
Inflammation is a prominent cause of secondary injury; SCI evokes substantially more potent neuroinflammation than brain injury [357] and elicits a multi-organ systemic reaction [45]. Post-SCI systemic inflammation includes damage to the lung, kidney [129], and liver [109, 273]. The extent of liver inflammation was greater for T4 as compared to T12 injuries. Other data supports that post-SCI immune dysfunction is spinal-level dependent and corresponds to the degree of loss of sympathetic regulation of the immune system [277]. The term “leukocyte” refers to all circulating white blood cells. Those known to be important in SCI are neutrophils, monocytes, and lymphocytes. Both leukocytes and monocytes independently contribute to injury propagation [201]. SCI leads to endothelial injury and the presentation of selectins and vascular cell adhesion protein-1 (VCAM-1) and intercellular adhesion molecule-1 (ICAM-1) which regulate monocyte rolling, stabilization, and diapedesis from the blood vessel into the perivascular space. Leukocytes express cell surface integrins that bind to the cell adhesion molecules (CAMs) and have roles both in endothelial adhesion and migration through vessel walls and tissue. This process mainly occurs in postcapillary venules where the shear forces are lower, fostering adhesion. Proof-of-concept studies have sought to deplete circulating white cells or to block their exit from the vascular space into spinal cord tissue. Depletion of circulating macrophages was associated with tissue preservation [30], improved locomotor outcomes [254], and reductions in fibrotic scar formation [362]. However, other studies that depleted neutrophils in mice found a worse outcome [307] indicating the complexity of the effects of inflammation after SCI and the limitations of simplistic approaches such as generalized blocking. Another study found that certain populations of macrophages that migrate into the injury site after SCI are neuroprotective [282] and that depletion of CD11-positive monocytes worsened neurological outcome. Others have shown that the persistence of macrophages is associated with axonal dieback [157]. Thus, the associated biology is complex.
Antibodies have been tested as therapeutics; those against P-selectin partially blocked neutrophil spinal tissue entry with reduced tissue myeloperoxidase activity and improved early locomotor function post-SCI [323]. CD11d (αDβ2 integrin) is expressed by monocytes and leukocytes and is involved in leukocyte activation and endothelial adhesion through interaction with its ligands ICAM-1 and VCAM-1 [28]. Mabon and colleagues developed a monoclonal antibody against αD to be administered intravenously in a transection rat model: 24 h before transection and 2 and 24 after transection (1 mg/kg dose). Two of the three anti-αD tested antibodies (228H and 236L) caused a 65 % reduction of macrophages in the injury site and one (236L) a 43 % reduction in neutrophils [219]. Later studies using a clip compression model assessed the mechanisms by which anti-CD11d was beneficial after SCI finding a reduction in myeloperoxidase (MPO) activity, thiobarbituric acid reactive substances (TBARS), and attenuated inducible nitric oxide synthase (iNOS) expression [16]. In another study, the reduction of reactive oxygen and nitrogen species was assessed demonstrating a decrease in COX-2 and 8-OHdG (a marker of RNA and DNA oxidation) expression, a reduction in protein carbonyl formation, and an increase in APE/Ref-1 (involved in repair of apurinic/apyrimidinic sites) formation [15]. Studies comparing anti-CD11d to MPSS showed both treatments increased neurofilament sparing, but myelin sparing was less with MPSS, with no differences in the neurological outcomes of the anti-CD11d + MPSS versus the MPSS group [339], but with significant differences in BBB scores when plotted against an anti-CD11d cohort reported by the researchers group in a previous publication [131]. There was no additive effect of MPSS; the anti-CD11d group had smaller lesion areas [130], increased serotoninergic fiber density below the injuries, and reduced allodynia in the dorsal trunk and hind paws [237].
Regarding therapeutic window, the treatment could be delayed until 6 h post-injury with improved BBB scores and decreased autonomic dysreflexia [79]. A subsequent study examined the relative expression of the key ligands on monocytes in normal adult humans versus those with general trauma and those with SCI. Higher densities of α4 and CD11d were found on neutrophils and monocytes after SCI but not after general trauma [13]. Anti-CD11d therapy also attenuated the systemic inflammatory response, with reduced inflammatory neutrophil and macrophage infiltration in lungs and kidneys [14]; this response was also evident after treatment with anti-α4β1 antibody [17]. Neuroprotective effects of anti-CD11d were found after SCI in mice [120]. An independent replication of the results previously reported was sponsored by the NIH to validate the results, but only nonsignificant trends on motor recovery and tissue sparing with the anti-CD11d treatments were found [161]. Another recent study used a different strategy to deplete both monocytes and neutrophils and found that the optimum protection required both be reduced [201]. Although there is substantial data in support of anti-CD11d antibody treatment post-SCI, no clinical trials have yet been announced. In stroke, an extensive study of an anti-ICAM antibody indicated the treatment worsened outcome [88] possibly due to the immunological effects of the use of murine antibody. Leukarrest, a humanized, anti- CD11b/18 antibody, reduced infarct size in animal models. However, no benefit was found in a phase III clinical trial [20].
20.4.3 Polyethylene Glycol
Polyethylene glycol (PEG) is a simple polymer with established safety in the pharmaceutical industry. It is a membrane fusogen, used, for example, in the generation of hybridoma cells to produce monoclonal antibodies [179]. Experiments conducted in a guinea pig severe spinal cord compression model showed that a solution of PEG in distilled water applied by pressure injection in the injury site repaired axons such that compound action potential conduction through the lesion recovered [283] and there was progressive recovery of the cutaneous trunci muscle reflex (CMT) [33]. Subcutaneous delivery of PEG was assessed and apparently selectively targeted the contused areas within the spinal cord with beneficial effects at a 6-h therapeutic window [32]. Histological three-dimensional reconstructions showed higher volumes of intact parenchyma and reduction of cystic cavitation in animals receiving PEG treatment directly over the spinal cord, immediately, or with a delay of 7 h after compression [84]. ROS elevation and lipid peroxidation also were inhibited by PEG [215]. A pilot study in dogs with SCI tested the intravenous delivery of the polymer at 30 % w/w in saline at 2 ml/kg within 72 h post-injury with a second delivery 4–6 h later. The authors reported no adverse events and an ambulation recovery in 68 % of treated dogs versus 25 % of controls and proprioception recovery in 42 % of treated versus 4 % of controls [197]. Mechanistic studies have indicated that PEG stabilizes mitochondrial calcium flux and reduces cytochrome-C release [211, 216, 217]. Additional rodent studies showed that animals treated within 15 min or 6 h with IV PEG had enhanced BBB scores and reduced mechanical allodynia up to a 6-week evaluation timepoint. When PEG and MgSO4 were combined, the additive effect was no greater than either single treatment [80]. An independent study conducted by Baptiste, Fehlings, and colleagues confirmed tissue-preserving effects of IV PEG 30 % delivered 15 min after 35-g clip compression. An increase in retrogradely labeled brainstem axons and a reduction in apoptosis of epicenter cells were observed. The small effects on locomotor scores left the authors to question if the therapeutic impact justified translation as a single treatment for SCI trials [18]. In a rodent contusion model, Kwon and colleagues assessed combinations of PEG with magnesium and found that PEG alone did not significantly improve open field locomotion up to 6 weeks posttreatment [186, 188]. More recent experiments performed by Borgens and colleagues include the incorporation of PEG with silica nanoparticles [55, 57] or micelles such as monomethoxy poly(ethylene glycol)-poly(d,L-lactic acid) di-block copolymer. Apparently, these formulations allow a more precise targeting to damaged neural tissue and a greater repair effect. It has been reported that PEG is superior to Matrigel, or alginate hydrogel in supporting regeneration after complete spinal transection [89]. So far PEG has not been studied in a human SCI clinical trial other than in combination with magnesium.
20.4.4 Estrogen
Clinical and experimental studies indicate that females tend to exhibit greater neurological recovery than males after equivalent spinal cord injuries [69, 96, 291], implicating a possible role for estrogen as a neuroprotectant. Estrogen has been shown to reduce leukocyte adhesion and infiltration [327] and attenuate microglial superoxide release and phagocytic activity [43]. In vitro experiments showed protection of hippocampal neurons against glucose deprivation, glutamate, FeSO4, and amyloid beta-peptide (Aβ) toxicity [126]. In 2004, Yune and colleagues reported neurological outcomes after a mild NYU contusion impact (12.5 g/cm) in male rats. 17β-estradiol was administered IV at 3, 100, or 300 μg/kg2 hours prior to injury or 100 μg/kg immediately post-injury. For the pre-administered doses, 100 μg/kg had the largest effect. After 30 days the BBB scores for animals in the 100 μg/kg cohort were 18.3 ± 0.6 (p < 0.001) versus 15 ± 1 in vehicle only. The lesion area was reduced, 2.06 ± 0.2 mm2 (p < 0.05) versus 2.5 ± 0.3 mm2, as was apoptosis 50 ± 5 versus 134 ± 8 (p < 0.001) TUNEL + ve, caspase-3 activation 5.7 ± 0.81 versus 9.8 ± 0.95, p < 0.001, and increased BCL-2 and BCL-X labeling. In another study cohort, the steroid was administered immediately after injury, and a substantial effect on BBB at 30 days was observed [351]. These data strongly supported a neuroprotective potential of 17β-estradiol in SCI but lacked clinical relevance since a therapeutic window was not assessed. Sribnick and colleagues reported in 2005 a male rat contusion model using higher doses of 17β-estradiol (4 mg/kg administered 15 min and 24 h post-injury IV). With a short survival of 48 h, there was reduction in tissue edema, infiltration of microglia and macrophages in the injury site and caudal penumbra, and attenuation of myelin loss in the injury site [300]. Further experiments by the group using the same methodology demonstrated attenuation in degradation of 68 kD neurofilament, reduction in calpain protein levels, decrease in cytochrome-C levels, and neuronal apoptosis in estrogen-treated animals [298, 299].
The issue of a neuroprotective effect of estrogen replacement in an SCI model of pre- and postmenopause tested the effect of preimplantation of a 17β-estradiol capsule (180 g/ml) 1 week before injury followed by a 0.5 mm forceps crush at T8/9. Neuroprotection was observed in both pre- and postmenopausal (ovariectomized) rats with improved white matter sparing, anterior horn cell preservation, reduced apoptosis, and higher BBB scores, although premenopausal rats (non-ovariectomized) had higher scores than estrogen treated. There was no effect on the manually voided urine volumes by 21 days post-injury [51]. Testing in the mouse species using a T10-controlled compression confirmed that females had substantially superior outcomes to males on the mouse BBB score [96].
A well-designed study tackled the issue of sex differences by comparing males to ovariectomized females with both implanted with a control, low-dose or high-dose estrogen-releasing capsule (low-dose 180 μg/ml, high-dose 1 mg/ml) 7 days prior to a moderate 150-kilodyne T10 injury. The serum estrogen levels were measured and reflected the dose delivery from the implanted capsule. The 21-day post-injury BBB was not significantly improved over the non-estrogen control in male or female rats. Injury length and tissue sparing favored females over males, but this effect was deemed not to be attributable to estrogen delivery. The authors concluded that no effect of estrogen delivery on SCI outcome was evident [318].
A mouse study used a 24-g clip compression at T6/7 with the subcutaneous delivery of 300–500 μg/kg of E2 before and after injury. They reported that estrogen reduced neutrophil infiltration, expression of myeloperoxidase, iNOS, nitrotyrosine, cyclooxygenase-2 (COX2), BAX, and apoptosis demonstrated by terminal deoxynucleotidyl transferase dUTP nick end labeling (TUNEL), TNF-α, IL-6, and IL-1β. The effects were abolished by a coadministered estrogen receptor antagonist [67]. Neuronal antiapoptotic effects and reduction in microglial activation were found in a rat contusion model even at small doses of 5 or 10 μg/kg [270]. A recent study showed that immediate post-T10 NYU 25 g/cm contusion delivery of 300 μg/kg of E2 protected the blood-brain barrier, with reduced hemorrhage, matrix metallopeptidase 9 (MMP9) and sulfonylurea receptor1 (Sur1) Transient receptor potential cation channel subfamily M member 4 (TrpM4) expression, reduced neutrophil and macrophage migration, and reduced inflammatory cytokine expression in male rats [199]. If estrogen is to be considered a potential neuroprotectant, there is a need for studies to establish a therapeutic window and rigorously evaluate safety.
20.4.5 Progesterone
Progesterone (PRO) is primarily known as female sex hormone produced by the ovary. However, PRO, like estrogen, has pleiotropic effects and is an important neurosteroid that is locally produced in the brain and has a key role in myelination [275]. PRO has been demonstrated to reduce inflammation and attenuate neuronal and glial loss [66, 303] in traumatic brain injury (TBI). Thomas and colleagues first reported testing progesterone therapy for SCI in a standard T9 25 g/cm NYU, contusion model. Adult male rats received 4 mg/kg of PRO intraperitoneally for 5 days beginning 30 min after injury, and significant improvements in BBB scores and white matter preservation were reported at the 6-week timepoint [328]. Fee et al. attempted to replicate some elements of the Thomas study but used a milder injury, 150-kilodyne T10 Infinite Horizon, and both male and female rats. PRO 4 mg/kg or 8 mg/kg was delivered at 30 min and 6 h post-injury and continued for either 5 or 14 days. As with the Kentucky group’s parallel study of estrogen [318], no gender differences were observed with PRO treatment after SCI. Regarding tissue sparing and BBB testing, there were no apparent differences effects between PRO and control at the 21-day assessment timepoint [98].
A recently published study evaluated PRO in male rats with a T8 200-kilodyne Infinite Horizon injury. Animals were randomized to receive subcutaneous injections of 16 mg/kg/day PRO or castor oil, starting 1 h post-injury and continued for 60 days until sacrifice. Outcome assessments included Catwalk automated gait analysis and stereological histological techniques. The authors found a benefit to PRO with improved BBB scores (mean 14 versus 11 in controls), subscores, and gait elements such as a reduced base of support and improved stride length and swing phase. Ex vivo MRI analysis found significant differences in injury volumes and spared tissue indicating a positive effect of PRO. There was a substantial sparing of white matter above and below the lesion epicenter. The number of CC1 immunopositive oligodendroglia, myelin basic protein (MBP)-positive segments, and neurofilament-labeled axonal profiles was increased in PRO-treated rats [114].
Other studies using a variety of injury models have identified several putative mechanisms of PRO effect. Reactive astrocytes in SCI were reduced in the injury site by doses of 4 mg/kg at 1, 24, 48, and 72 h post-T10 transection in male rats [193]. The same treatment paradigm restored the expression of the α3 and β1 regulatory subunits of NA-K-ATPase with further upregulation of growth-associated protein (GAP-43) in ventral horn motoneurons [190], produced upregulation of NG2-positive cells [70], enhanced brain derived neurotrophic factor (BDNF) immunoreactivity, and prevented chromatolytic degeneration in motoneurons with preservation of the cytoskeletal component identified by MAP2 [124, 125]. Regarding potential effects on myelination, a dose of 16 mg/kg started 3 h post-injury and continued until sacrifice increased the expression of mRNA and MBP and increased mRNA and protein expression for oligodendrocyte differentiation factors Olig2 and Nkx2.2 at 3 days. If treatment was continued until 21 days, markers of mature myelin recovery included reduced oligodendrocyte precursor cells and increased oligodendrocyte mRNA expression of proteolipid protein (PLP) and Olig1 [191]. In a different study, inhibition of astrocyte and microglia/macrophage proliferation and activation was found [192]. Currently, there is no indication for the use of progesterone in the clinic as a neuroprotective for SCI, and further study of a therapeutic window in contusion injury would be important.
20.4.6 Nonsteroidal Anti-inflammatory Drugs (NSAIDs)
Another important class of anti-inflammatory drugs for which there is extensive clinical experience are NSAIDs that inhibit cyclooxygenase 1 and 2 and thus the production of eicosanoids from arachidonic acid. Their activity reduces the production of prostaglandins, thromboxanes, and leukotrienes. Pre-injury delivery of indomethacin substantially reduced tissue prostaglandin levels in a feline SCI model [172]. In another feline SCI study, a triple combination treatment of indomethacin, heparin, and prostacyclin (PGI2) one and three hours post-weight-drop contusion was compared to naloxone and saline control. The combination including indomethacin showed similar Tarlov locomotor scores to those animals receiving naloxone, with both superior to saline control [144]. Fujita and colleagues found that aspirin pretreatment (5–50 mg/kg) also reduced lipid peroxidation and decreased prostaglandin production [111]. A dual lipoxygenase/cyclooxygenase inhibitor BW755C administered prior to trauma decreased the accumulation of thromboxane B2, improving neurological recovery in rat contusion model, but the same effect was not achieved when applied post-injury [93].
Further experimental studies conducted during the 1990s assessed the effects of indomethacin after SCI. These showed neurological improvement with reduced tissue injury in rats (2 mg/kg post-injury + every 24 h during 7 days) [290], decreased edema and attenuated motor evoked potential changes (10 mg/kg 30 min pre-injury) [342], and ultrastructural demonstration of decreased edema (10 mg/kg 30 min pre-injury) [279].
A lack of effect of indomethacin was also reported: no locomotor improvement unless combined with pregnenolone and lipopolysaccharide (0.2 mg after injury + every 24h during 21 days) [138], increased lipid peroxidation when compared to saline treatment (3 mg/kg after injury) [139], and increased levels of MPO and TNF with greater motor deficit and histological damage (5, 10, 20 mg/kg 1 h pre-injury) [149]. The study by Guth and colleagues is notable as this was a combination selected to increase monocyte cytokine secretion using lipopolysaccharide, to provide anti-inflammatory steroid activity with pregnenolone, and to block prostaglandin production with indomethacin. Multiple pair-wise and triple combinations were tested that included aspirin and dehydroepiandrosteron (DHEAS) as alternate anti-inflammatory controls. The injury was created at T8 using 2 s of jeweler’s forceps compression. Only indomethacin, pregnenolone, and lipopolysaccharide (LPS) combined were associated with substantial locomotor recovery and reduction in the lesion area. Eighteen years later, an National Institute of Neurological Disorders and Stroke (NINDS) supported replication was reported by the team at Ohio State. An attempt was made to replicate the forceps compression injury, and a sustained release pellet (lipopolysaccharide 0.2 mg, indomethacin 0.16 mg, pregnenolone 150 mg) was inserted into tissue adjacent to the laminectomy, and the animals survived for 28 days. An effect of the triple combination was found on white matter preservation, preserved neurofilament area, and axon density. No effect, however, was detected in the BBB scores, subscores, or imputed Tarlov scores, with assessments up to 28 days post-injury [256]. In a brief rebuttal, Dr. Guth pointed out that the control animals in the replication study showed more spontaneous recovery than in the original study making it difficult to show a difference in locomotor recovery [137].
A very interesting property of some NSAIDs is the ability to reduce RhoA activation after SCI. In the first study, indomethacin was injected at 2 mg/kg/day IP after a T8 dorsal over hemisection. The tissue was examined on day 3 for sources of RhoA activity. The treated rats showed a decrease in RhoA expression in microglia/macrophages, astrocytes, and neutrophils with an increase in GAP-43 positive neurites [276]. In a second experiment, the impact of ibuprofen versus naproxen on RhoA activation, axonal growth, and locomotor recovery was assessed, with drugs delivered for 28 days via an Alzet minipump, ibuprofen (60 mg/kg/day), naproxen (50 mg/kg/day), or saline. The treatment was initiated immediately after an extensive dorsal T6/7 oversection, or 7 days after a T8/9 moderate NYU contusion injury. In vitro assays showed inhibition of RhoA activation by ibuprofen and indomethacin but not naprosyn. Interestingly, the inhibition was of similar potency to C3 transferase (see Cethrin above). At 5–7 days post-contusion, RhoA was markedly reduced in spinal cord tissue by ibuprofen but not naprosyn. In the sharp section, animals with immediate delivery CST axon growth distal to the lesion were found in anterogradely traced animals that received ibuprofen but not naprosyn or control. In the contusion animals with treatment initiated at 7 days post-SCI, improved serotonergic axonal growth below the injury level was observed. In both injury paradigms, the BBB score at 42 days was higher in the ibuprofen-treated animals [110]. A subsequent study further examined these issues using both in vitro assays and rat experiments. A T7/8 25 g/cm NYU contusion was created and 3 days after injury an Alzet minipump implanted to deliver 70 mg/kg/day ibuprofen, 25 mg/kg/day naproxen, or PBS. The rats survived either 2 or 6.5 weeks. In a separate experiment, mice underwent T8 spinal cord transection and were simultaneously implanted with minipumps to deliver either 35 or 70 mg/kg/day of ibuprofen for 28 days. The in vitro studies showed a dose-dependent reduction in MAG-mediated inhibition. Both weight-bearing status and BBB scores were increasing in ibuprofen- but not naprosyn-treated animals up to 50 days post-injury, and Rho activation was reduced in spinal cord tissue. Tissue preservation was significantly better in ibuprofen as compared to naprosyn- and PBS-treated animals at 2- and 4-week timepoints. There was extensive sprouting of the CST rostral to the contusion site in contused rats that received ibuprofen, and the terminations of the tract were not retracted from the injury as is usually seen and was observed in the naprosyn- and PBS-treated animals. No distal CST regeneration, however, was observed. Six weeks post-injury, ibuprofen-treated rats showed substantially more serotonergic fiber density distal to the contusion site, apparently due to regenerative sprouting from preserved processes. In completely transected mice, regenerative growth of serotonergic fibers through the transection and distally was observed together with improvements in the Basso Mouse Scale for Locomotion (BMS) [337]. The Fu et al. study was selected for replication by the Facilities of Research Excellence—Spinal Cord Injury (FORE-SCI) NIH group, conducted at UC Irvine. Despite consultation with the senior author, Dr. Li, of the index study, no aspect of the original results could be confirmed other than the suppression of Rho activation by ibuprofen [280]. A recently reported study assessed ibuprofen in combination with C16, a vascular preserving peptide; ghrelin, a neuroprotective gastric hormone; and ketogenic diet in a C5 hemicontusion paradigm. No effects were apparent using rodent upper extremity functional assessments and light microscopy-based tissue sparing analysis [309].
Selective inhibitors of inducible cyclooxygenase 2 were studied. Following a 25-g/cm NYU impact, celebocid (3 mg/kg) was delivered PO 20 min post-injury. The levels of prostaglandin E2 (PGE2) and thromboxane B2 (TxB2) were reduced by the drug at 4 and 24 h post-injury [264]. A single injection of NS-398 5 mg/kg delivered 15 min prior to a 12.5 g/cm NYU T13 also decreased prostaglandin E2 (PGE2), increased spared tissue, improved BBB scores 14–28 days post-SCI, and reduced mechanical allodynia and thermal hyperalgesia [141]. It is notable that in this injury model, the pain behaviors are found in forelimbs above the injury and the hindlimbs below the injury. This indicates that CNS reorganization after SCI occurs not only at and below the injury level.
20.4.7 Glibenclamide
Glibenclamide, known as glyburide in the USA, is a well-known sulfonylurea developed in the 1960s for the oral treatment of type 2 diabetes [226]. Due to its ability to close KATP channels (pore formed by subunits SUR1 and Kir6.2), it stimulates the secretion of insulin by β islet cells [10]. Interest in its potential for SCI was due to the identification of an upregulation of the SUR1 subunit in a characteristic phenomenon of TBI and SCI known as progressive hemorrhagic necrosis (PHN) [285], which is described as a progressively confluent petechial hemorrhage adjacent to the injury sites [12]. The mechanism of PHN is not yet known, but it is understood to be a progressive capillary blocking due to mechanical stress after secondary injury [325] or leukocyte infiltration [175].
In a rat cervical hemicontusion model, SUR1 was identified to be upregulated in endothelial cells and neurons surrounding necrotic injuries, beginning its expression in the epicenter at 6 h post-injury and expanding to the injury rim and adjacent tissues within 24 h. The delivery of glibenclamide via infusion pump (200 ng/h) after 2–3 min of injury resulted in a threefold reduction of injury volumes; decreased intraspinal hemorrhage at 6, 12, and 24 h; and significant neurobehavioral improvement in BBB scores and inclined plane testing at 1, 3, and 7 days post-injury [288]. An NIH-sponsored independent replication study was carefully conducted. When initial data failed to replicate the index study of Simard and colleagues, extensive efforts were made to ensure that the same injury methodology was being employed. When this was achieved, an effect of glibenclamide could be verified [255, 256]. This experience underscores the importance of exact attention to detail in replication studies. In a subsequent study, the effect of glibenclamide was assessed in two hemicontusion injury methods. In the more extensive injury, small but significant effects of glibenclamide on behavioral outcomes and tissue sparing were observed [287]. It should be noted, however, that the drug was administered within 5 min of injury. In another study, glibenclamide was compared to riluzole with administration performed either immediately after unilateral C7 injury or at the 3-h timepoint. Unfortunately, nearly all of the control animals died resulting in a comparison between the two drugs but without a vehicle comparison. Glibenclamide resulted in superior tissue preservation, grip strength, rotarod stability, and rearing [289]. A subsequent study assessed the effects of glibenclamide on the progression of hemorrhagic injury volume using MRI [286]. Glibenclamide appears as a promising neuroprotective approach for SCI, but further studies in more severe lesion models and with additional timepoints of drug delivery should be conducted to compare its effects to other agents in rodent models. Clinical trials in stroke and TBI have been initiated.
20.4.8 Thiazolidinediones
Thiazolidinediones (TZDs) are used in the treatment of type 2 diabetes and are agonists of the peroxisome proliferator-activated receptors gamma (PPARγ). These are “nuclear receptor” DNA-binding compounds that regulate fatty acid storage and hepatic glucose metabolism [170]. A model assessing factors involved in the macrophage-mediated expansion of SCI cavity size included testing of ciglitazone (the prototypical TZD) with an in vitro cavity model of cultured astrocytes and activated macrophages. Ciglitazone potently reduced the increase in the areas of culture cavities mediated by zymogen-activated macrophages [106]. Later with the development of pioglitazone (PGZ) which crosses the blood-brain barrier [317], a rat contusion model compared high (10 mg/kg) versus low (1 mg/kg) doses of the TZD, started 15 min post-injury and maintained BID for 7 days. The differences in BBB scores were not significant with a positive trend for the high-dose group (15.4 ± 1.1 versus 13.3 ± 1.3 in controls), but differences were more evident in subscores (toe clearance, coordination, parallel paw position). The high-dose group had improvements in spared white and gray matter areas rostral to the epicenter and better neuronal counts rostral and caudal to the epicenter; no differences were observed in caspase-3 immunoreactivity or macrophage detection [232]. A concurrent study by a different laboratory compared the treatment effects of PGZ and rosiglitazone (RGZ) (0.5, 1.5, 3 mg/kg at different timepoints) and the PPARγ antagonist GW9662 (2 mg/kg 1 h prior to any TZD injection). Both TZDs decreased lesion areas (57 % RGZ, 64 % PGZ) significantly and increased neuronal survival; decreased astrogliosis, microglial activation, myelin loss, induction of inflammatory genes; and enhanced induction of neuroprotective heat-shock proteins and antioxidants, with improved BBB scores (final scores at day 7 PGZ 12.9 ± 1.4, RGZ 12.2 ± 1.1, vehicle 8.9 ± 0.7); these effects were inhibited by the GW9662 treatment. The therapeutic window of intervention indicated no significant effects of a delay in administration >2 h and similar effects with 3 or 1.5 mg doses with no effects at 0.5 mg; additionally PGZ exhibited a decrease in thermal hyperalgesia [244]. In other studies, intrathecal delivery of RGZ decreased neuropathic pain [59], and similar effects were observed with oral administration of PGZ [220]. Anti-inflammatory effects of RGZ (reduction of TNF-α, IL-β, myeloperoxidase activity) were evident in a clip compression model [358], and a comparison between MPSS and RGZ demonstrated greater increased neurotrophin expression and improved early recovery in the RGZ group [359]. There are no published studies in SCI to disprove the effects of TZD treatment, and having two studies performed by independent groups with similar results within the same year is strong evidence. There are additional reports from Chinese studies [169, 207, 345]. It may be valuable to test TZD in a large animal contusion model.
20.4.9 Atorvastatin
Atorvastatin and statins, in general, are competitive inhibitors of the 3-hydroxy-3methylglutarylcoenzymeA (HMG-CoA) reductase which catalyzes the reduction of HMG-CoA to mevalonate, inhibiting the synthesis of hepatic cholesterol, increasing the uptake of low density lipoprotein (LDL), reducing triglyceride levels, and increasing high density lipoprotein (HDL) levels. Statins have anti-inflammatory effects, in astrocyte and microglial cell cultures exposed to LPS. Lovastatin blocked the expression of inducible nitric oxide synthase (NOS) and TNF-α, IL-1β, and IL-6 [241]. In MBP-induced experimental models of experimental autoimmune encephalomyelitis (EAE) in Lewis rats, lovastatin was found to reduce the numbers of infiltrating mononuclear cells by downregulating the ICAM ligand Lymphocyte function-associated antigen-1 (LFA-1), to reduce demyelination, and to reverse paralysis [301]. In further EAE studies, atorvastatin was found to increase a Th2 over Th1 cytokine and cellular phenotype [350]. The studies in SCI by Pannu and colleagues used a rat contusion model with an oral atorvastatin dose of 5 mg/kg/day until sacrifice (15 days). All groups in the study received a pretreatment regimen of atorvastatin for 7 days; animals that continued on statins exhibited higher BBB scores that started to deviate significantly from the vehicle group by day 3 (6.57 ± 0.55 vs 0.166 ± 0.33) and showed near-normal scores by day 15 (19.13 ± 0.53 vs 9.04 ± 1.22). Statin-treated animals had reduced post-injury levels of TNF-α and IL-1β, decreased infiltration of ED-1 macrophages, attenuated neuron, and oligodendrocyte apoptosis demonstrated by TUNEL assay, reduced gliosis, tissue necrosis, and demyelination [242]. Evaluation of the therapeutic window was subsequently assessed by not pretreating and randomizing contused animals to start treatment at 2, 4, or 6 h post-injury, with continued drug treatment until sacrifice (42 days). BBB analysis showed much higher scores for all treated groups at 42 days (2h 19.5 ± 1, 4h 19.25 ± 1, 18.5 ± 0.5) versus 10.5 ± 1.5 vehicle control; these results were supported by attenuated necrosis and demyelination; decreased neutrophil and macrophage infiltration; decreased expression of iNOS, TNF-α, and IL-1β; attenuated expression of MMP9 via inhibition of the RhoA/ROCK pathway with preservation of the blood-brain barrier; and reduced neuronal apoptosis [243]. These are remarkable results clearly requiring independent evaluation. A Canadian study assessed the relative effects of simvastatin and atorvastatin as the former is more lipophilic, using oral gavage delivery in saline, fed in chocolate ensure, or purified simvastatin alone administered subcutaneously initiated 2h after injury. The OSU device was used to deliver a 1.5-cm displacement at 300 m/s. In brief, the results failed to show effects that justify clinical translation. There were higher BBB scores in the statin group in one experiment that could not be reproduced in others: white matter tissue sparing showed a nonsignificant trend toward positive effects but did not reach statistical significance (42 ± 6% vs. 34 ± 8% in controls), and the one statistically significant difference was obtained at 3 days comparing the extent of ED1 staining at the injury epicenter [224]. A second Canadian study examined the effect of atorvastatin-administered IP 5 mg/kg 2 h post-SCI for 15d with a 4 week survival. An early reduction in TUNEL labeling and caspase-3 activation was observed, with a statistically significant improvement in BBB score at 4 weeks post-SCI. In conclusion, statins may offer an approach for post-SCI neuroprotection, but additional evidence is needed to resolve inconsistencies in the literature and determine if the cost and effort of a clinical trial is warranted.
20.4.10 Inosine
Inosine is a purine nucleoside found in tRNAs and is a popular athletic supplement. It’s metabolism to uric acid has antioxidant effects. One of its earliest uses in neurology was as a component of the drug isoprinosine that was found to have beneficial immune-modulating properties during viral diseases such as subacute sclerosing panencephalitis [171]. Inosine stimulated axonal outgrowth in retinal ganglion cell cultures [26]. Subsequently, its impact on CST structural plasticity was studied in a rodent model of unilateral pyramidal section. Twenty-four hours after the injury, animals were implanted with minipumps to continuously infuse inosine (10 mM 0.5 μl/h) for 14 days into the non-axotomized sensorimotor cortex. Inosine stimulated the uninjured pyramidal axons below the lesion to extend new collaterals across the midline into denervated areas of the lesioned tract [24]. An upregulation of GAP-43 expression was observed in axons forming new collaterals [25] as is seen in regenerating axons. Mammalian sterile 20-like kinase-3b (Mst3b) activation was specifically linked to inosine stimulated axon outgrowth [165]. Further studies have indicated that Mst3b is an important regulator of axonal growth after injury in both CNS and peripheral nervous system (PNS) neurons [165, 213]. In models of unilateral stroke, intraventricular delivery of inosine [354] did not reduce infarct size but was associated with improved recovery of affected forepaw function, sprouting of CST axons into the denervated tract, and upregulation of genes associated with axonal growth in the uninjured cortex. In a second stroke study, inosine amplified the effects of a co-administered Nogo blocker and was again associated with increased forelimb function [353]. In a rodent T8 dorsal spinal cord hemisection model, the effects of intraventricular (50 mM 0.5 μl/h) and intravenous (8–27 mg/kg/day) inosine delivery were compared. Both delivery methods were associated with significant increases in BBB scores and ladder walking tests. Inosine treatment did not affect lesion size but increased CST sprouting in the cervical spinal cord rostral to the lesion with new synaptic contacts with long propriospinal interneurons that could form novel relays and also was associated with increased serotoninergic immunoreactivity to the lumbar spinal cord below the cord section [176]. In a Chinese study, a single dose of inosine was administered IP at 2, 12, or 24 h after T9 clip compression injury. Epicenter tissue preservation and a reduction in the number of apoptotic cells were detected at 3 days in the 2- and 12-h treatment groups with the 2-h treatment being the best [210]. In another experiment, inosine effects appeared to be enhanced by oscillating field stimulation with regeneration of ascending and descending projections of spinal cord tracts and recovery of the cutaneous trunci muscle reflex in chronic SCI [31]. Continued subcutaneous delivery of inosine started 15 min post-thoracic contusion injury reduced the number of ED-1 positive profiles at the injury site [62]. In 2012, an NIH-sponsored independent replication study of the methodology published by Benowitz in 1999 was reported from UC Irvine. The authors described difficulties with complications and deaths secondary to the pyramidotomy surgeries; nevertheless, after several trials the results failed to confirm that CST axons sprouted across the midline [304, 305]. Sources of possible error in interpreting the histological appearance of CST axons at the midline were discussed. The most recent study testing inosine in SCI reported neuronal survival, tissue sparing, and improved locomotion after oral administration of inosine (1.2 g/kg/day, beginning 2 h post-injury) [185]. In summary, there is substantial evidence for inosine as a promoter of axonal plasticity after injury. Combined with its established use in drugs and as a health supplement, clinical testing may be reasonable if pivotal preclinical studies to assess the route of administration and pharmacokinetics were supportive.
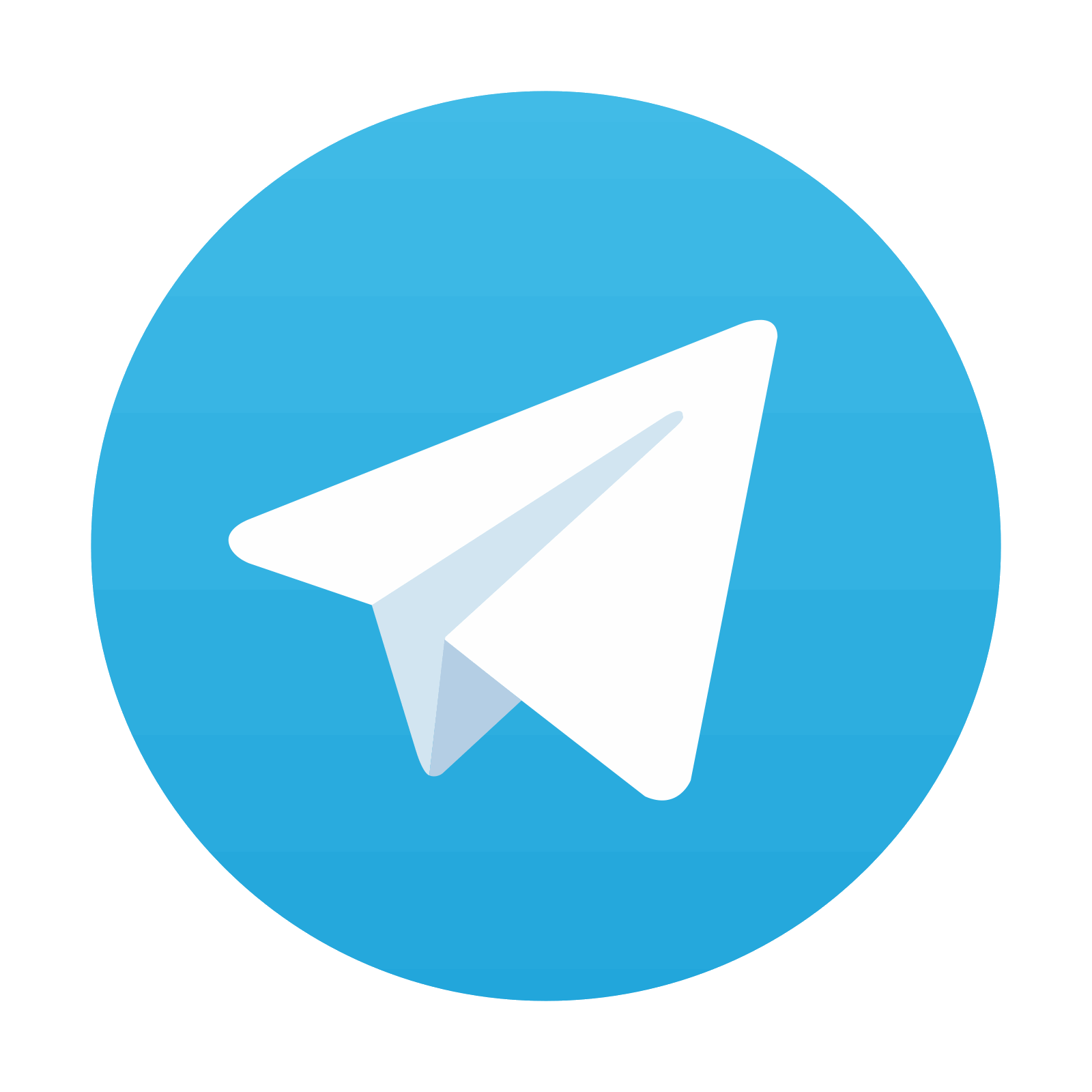
Stay updated, free articles. Join our Telegram channel
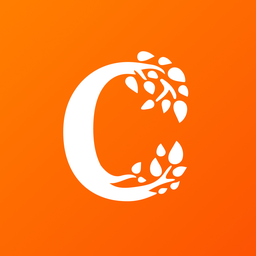
Full access? Get Clinical Tree
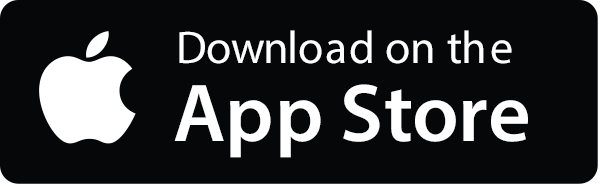
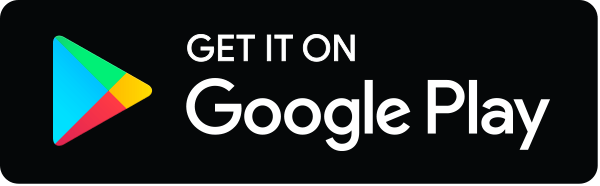