The Cartilage–Bone Interface
All Cartilage–Bone Interfaces Are Derived from an Initially Pure Cartilage Structure
Long bones develop first from embryonic mesenchymal stem cells that coalesce to form a “blastema,” with a scant but uniform type I collagen matrix.1 The blastema transforms at early fetal stages into a cartilaginous structure, or cartilage “anlage,” with collagen type II as the main extracellular component.2–6 The cartilage anlage contains a mixture of fusi-form and round chondroblast cells,7 which upon terminal differentiation will hypertrophy (become unusually large) and begin to express proteins that attract blood vessels and facilitate biomineralization.8
Mineralized bone begins to form when the fetal cartilage undergoes focal hyper-trophy, which launches a process of endochondral ossification (EO). The very first cartilage–bone interfaces to form in the body are at the primary ossification centers in the shaft of developing long bones. These areas are marked by remodeling and vascular invasion in parallel with the deposition of a mineralizing collagen type I matrix that ensheathes and mechanically protects the blood vessels.4,9 After the secondary ossification centers appear in the distal tibia and femur, three types of dynamic cartilage–bone interface are established, as illustrated in the immature rabbit knee ( Fig. 5.1a–c ). Articular cartilage sits on top of the epiphy-seal subchondral bone ( Fig. 5.1b, e, h ). The growth plate is a cartilage structure firmly sandwiched between two layers of bone: the epiphyseal and the metaphyseal bone ( Fig. 5.1c, f, i ).
Growth Plate Cartilage–Bone Interface during Postnatal Development
In the developing knee, epiphyseal bone will continue to expand into the cartilage anlage until the cartilage interface forms a thin calcified layer that arrests vascular invasion. Calcified cartilage forms at the base of the articular cartilage and in certain growth plate reserve zones ( Fig. 5.2 ), through mechanisms that are still not fully understood. Haines10 previously noticed that growth plate reserve zones fused to “permanent” epiphyseal lines develop a thin layer of calcified cartilage/tidemark ( Fig. 5.2a , proximal trochlea) while other reserve zones do not form tidemarks ( Fig. 5.2b , distal trochlea) and eventually close without leaving a scar.
Growth plate hypertrophic cartilage (HTC) does not form a tidemark. This interface is actually a mixture of cartilage and bone, by definition of the primary spongiosa, where new bone is deposited on the cartilage trabeculae carved out by invading blood vessels and marrow ( Figs. 5.1f, i, and 5.3 ). In trabecular bone maturing below the growth plate, an initially pure collagen type II-glycosaminoglycan (GAG) extracellular matrix is slowly incorporating collagen type I (EO, Fig. 5.3 ). Vascular invasion of the hypertrophic zone spurs a continual endochondral expansion of the distal femur (arrows, Fig. 5.4a ), in tandem with appositional cartilage and bone growth ( Fig. 5.4b, c ).

The growth cartilage–metaphyseal bone interface is a dynamic and ever-expanding front of HTC undergoing vascular invasion and ossification. Interestingly, in newly formed endochondral bone, hypertrophic chondrocytes express zymogen forms of enzymes capable of remodeling collagen matrix, including matrix metalloproteinases (MMP-13, MMP-9) and complement C1s.11,12 In knockout mice for MMP-13 or MMP-9, conversion of collagen type II HTC to collagen type I trabecular bone is inhibited.13–15 Remodeling of the HTC front by osteoclasts, chondroclasts, and bone-marrow-derived metalloproteinases drives the replacement of HTC with vascularized bone.13–15 Growth plate cell proliferation and vascular invasion can be diminished by nutritional deprivation, ischemia, or supraphysiologic loading.16–18 Blood vessel invasion of the HTC layer is believed to be naturally driven by hyper-trophic chondrocyte secretion of angiogenic factors,19 MMP-13,11,13 and gelatinases capable of untethering matrix-bound vascular endothelial growth factor.15 Osteoclasts that remodel the base of endochondral bone are also known to release angiogenic factors and can also promote vascular invasion and osteogenesis.20,21 Apoptosis of hypertrophic chondrocytes is also implicated as an important driver of the endochondral growth process.15,22

Articular Cartilage–Bone Interface during Postnatal Development
A distinct and more advanced EO process is going on during postnatal articular cartilage growth ( Fig. 5.1e and h ). In 3- to 6-month-old rabbit articular cartilage, most chondrocytes are no longer proliferating, and a tidemark has formed at the base of the hypertrophic zone.23 Bone is not being deposited along cartilage trabeculae; it has developed layer by layer to form a thick osteoid around blood vessels subjacent to the calcified cartilage layer. Only small patches of cartilage persist in the subchondral bone (EO, Fig. 5.1e ). The remnants of GAG and collagen type II in trabecular bone are the hallmarks of EO.
Neonatal articular cartilage is relatively thick; it is filled with a system of endothelial-lined canals distinct from the normal vasculature.7 Cartilage canals have been described in immature articular cartilage in a variety of large animals and in human (fetal ovine, 2-week-old calf, 2-year-old human).7,24 Postnatal weight-bearing activity is associated with regression of the canals and a thinning and anisotropic organization of the articular cartilage layer. The articular layer continues to grow postnatally through an appositional or asymmetric layer-by-layer expansion, through cell division near the superficial zone6,25,26 ( Fig. 5.4 ). In the deep zone near the articular cartilage–bone interface, chondrocytes terminally differentiate into hypertrophic chondrocytes, cease to proliferate, and express collagen type II, collagen type X, alkaline phosphatase, and osteopontin, a highly phosphorylated hydroxylapatite-binding protein.27–31 Like articular cartilage, the growth plate hypertrophic zone also contains collagen type X and alkaline phosphatase, but a tidemark is notably absent.32,33 The tidemark that forms at the base of mature articular cartilage develops slightly below the region of chondrocytes expressing collagen type X.28 Mineral deposits form in the neonatal calcified layer of the articular cartilage in line with the collagen fibers.34 Using fluorescent pulse labeling of the mineral phase, Oegema et al observed that the tidemark advances above the pulse-labeled mineralization front in 4-month-old rabbit patella at a rate of 8 µm/week, compared with 1 µm/week in the 7-month-old rabbit patella.26 Creeping advancement of the tidemark is associated with thinning of the articular cartilage layer.23

Vascular channels are branched structures that supply the calcified cartilage of the articular layer7,9,35 and have similarities with vascular channels in the vertebral endplate where bone abuts the cartilaginous nucleus pulposus.36 The calcified cartilage zone is thus normally vascularized, whereas the nonmineralized cartilage above the tide-mark is normally avascular. In a study by Bonde et al,37 blood vessels were observed to only sporadically penetrate the tidemark into cartilage in normal patellar cadaveric subjects (less than one average blood vessel per normal patella from subjects 75 to 89 years old) compared with an average of nine tidemark-penetrating vessels per subject in osteoarthritis (OA) femoral condyle samples. Pathologic blood vessel passage beyond the tidemark is associated with occasional thrombosis, a thicker calcified cartilage layer, and tidemark duplication37 ( Table 5.1 ).
The calcified cartilage layer is semipermeable and permits passage of small molecules (<500 Da) from the subchondral bone to the articular cartilage layer.38,39 Conversely, immersion of a mouse distal femur end in fluorescein allows full solute passage through the articular cartilage and selective fluorescein diffusion into chondrocytes in the calcified cartilage layer.39 Calcified cartilage permeability was measured as fivefold less than noncalcified cartilage in mature horse metacarpal tissues.38 It has been hypothesized that venous congestion in the synovium and subchondral bone could play a role in tidemark duplication.37 Thickening of the calcified cartilage in OA could be expected to reduce the flow of small solutes from the vascularized subchondral bone to the deep zone chondrocytes.

Once formed, the tidemark and calcified cartilage layer persist as dynamic structures that can change and remodel over time. Below mature articular cartilage, the mineralization front is a relatively smooth and undulating plate-like surface, as illustrated in a microcomputed tomography (micro-CT) 3D image of the calcified cartilage and bone below the trochlear articular cartilage in a 30-month-old rabbit knee ( Fig. 5.5a ). The tidemark at this stage is a strong hematoxylin-stained line ( Fig. 5.5b ). Some remnant or newly duplicating tidemarks can be observed within the mature calcified cartilage layer (open arrowheads, Fig. 5.5b ). In the skeletally immature 4-month-old rabbit, a more irregular mineralization front is observed at the articular cartilage–bone interface, which corresponds to the subchondral bone and a thin layer of calcified cartilage ( Fig. 5.5c ). At this stage a nascent tidemark can be visualized using a hematoxylin-eosin stain, and the vascular bone channels are just below, with close communication between the vasculature and calcified articular cartilage ( Fig. 5.5d ). By contrast, in the same 4-month-old rabbit trochlear specimen, the growth plate HTC contains a highly irregular and discontinuous mineralization front at the growing bone–cartilage interface with no visible tidemark ( Fig. 5.5e, f ). The mineral front at the base of the growth plate corresponds with the vascular bone and newly deposited collagen type I (black arrowheads, Fig. 5.5f ). In the growth plate hypertrophic zone, calcification of the collagen type II matrix is much delayed compared with the articular cartilage calcified layer. This is because after birth the mammalian joints require a suitable mechanically stable articular surface, while growth plates in the long bones are continually expanding, even beyond sexual maturity. Cartilage calcification is therefore occurring only at the end-stage of cartilage growth. After reaching skeletal maturity, growth plates are completely resorbed and replaced by collagen type I–positive mineralized bone.
To summarize, growth plates develop a relatively stable reserve zone–epiphyseal bone interface, with a purely collagen type II GAG-rich cartilage phase and a mixture of collagen type I and collagen type II in the newly forming primary spongiosa. Calcified cartilage becomes established at the edges of a “permanent” epiphyseal bone layer (i.e., proximal reserve zone and articular cartilage hypertrophic zone), and the tidemark serves as a barrier to vascular invasion and calcification of hyaline cartilage.
Structure and Mineral Content of the Mature Articular Cartilage–Bone Interface
Articular calcified cartilage is a mineralized layer in which extracellular matrix is chiefly composed of collagen type II, collagen type X, and GAG19; the layer also contains extracellular alkaline phosphatase.31 Alkaline phosphatase can generate free phosphate from organophosphates such as β-glycerol phosphate, for incorporation into hydroxylapatite mineral (Ca-P).40–42 In the calcified cartilage layer of normal human femoral condyles, chondrocytes are quiescent and present at a much lower density compared with hya-line cartilage (average of 51 cells/mm2 vs. 152 cells/mm2).43 The calcified cartilage layer is flanked by an undulating tidemark, and an even more irregular cement line adjacent to the bone. Wang et al43 analyzed normal adult human bone (20- to 45-year-old cadaveric) by histomorphometry and stereology to show that hyaline cartilage is interlocked tightly in a “ravine-engomphosis” structure with the calcified cartilage zone, which is then attached in a “comb-anchor” to bone.43 The surface roughness was determined to be 1.14 (tidemark) and 1.99 (cement line).43 The more irregular cement line–bone interface is the end result of an inhomogeneous vascular invasion during development of the calcified cartilage layer (white arrowheads, Fig. 5.1h ).

In normal human subjects, the mean calcified cartilage thickness is variable, from 20 m to ∼ 250 µm.44–46 The calcified cartilage is tightly fused to the articular cartilage and subchondral bone plate composed of lamellar bone, along with punctate regions where the calcified cartilage is in direct contact with vascular channels.7,47 In any group of individuals, the mean calcified cartilage thickness and mineral density will vary according to age, site in the joint, and mechanical loading ( Table 5.1 ).44,48,49 In a study of normal femoral head cadaveric specimens with no signs of OA by Lane and Bullough,44 the calcified cartilage thickness in the femoral head varied from 79 µm to 243 µm, with a thicker calcified cartilage in less stressed areas of the hip joint. Müller-Gerbl et al45 performed a similar study in normal cadaveric femur heads and found the calcified cartilage thickness varied from 20 m to 230 m, and that the ratio of calcified cartilage to total cartilage thickness was relatively constant. The calcified cartilage layer shows gradual thinning with age, along with tidemark duplication in subjects over 70 years old (see Table 5.1 ).44 Lane and Bullough concluded that the calcified layer is undergoing continual resorption and endochondral advancement over time.44 These observations are consistent with the measured dwindling rate of advancement of the tidemark with age in rabbit patella.26 In 31 normal human femoral condyles, an age-dependent loss in bone mass was measured in the subchondral bone plate.50 The bone volume fraction (bone volume/total volume%) of the bone plate region was observed to decline from ∼ 36% for subjects in their 20s to ∼ 27% for those >80 years old.50 Bone loss was attributed to thinning of the subchondral trabeculae with age (as opposed to diminished trabecular number), and this occurred at a relatively steady rate (Trabecular Thickness = 141 µm 0.63 × age).50 By contrast, in OA, a pathological increase in the calcified cartilage layer thickness arises, along with abnormal tidemark duplication37 (see Bonde et al, Table 5.1 ), and this is frequently accompanied by subchondral bone plate thickening and sclerosis.51 The consequence or implication of tidemark duplication is not known, although Burr has proposed that microcracks at the bone–cartilage interface may be implicated in the etiology.51
The mineral component in the calcified cartilage layer is similar but distinct from that found in bone, and notably influenced by the uniform presence of GAG. In a study by Rey et al, pulverized calcifying cartilage from 2-month-old calves (collagen type II-positive and type I-negative) had a very low mineral content (2.8% by weight), with an immature, very poorly crystalline and low carbonate apatite mineral [calcium/(phosphate + carbonate)], compared with bone (normally ∼ 0.20 carbonate/P).41 The calcified cartilage mineral phase was also characterized by a large proportion of nonapatite “brushite-like” phosphate.41 Unlike bone, this high nonmineral, labile phosphate content actually increases over time.41 The low mineral content measured in immature calcified cartilage by Rey et al41 is consistent with the quite irregular mineral surface of the epiphy-seal growth plate shown in Fig. 5.5c . In this sample, the mineral surface most probably corresponds to mineralized collagen type I because the threshold level used in this three-dimensional reconstruction model would remove hypomineralized calcified cartilage from the image (i.e., areas between the black arrows and black arrowheads, Fig. 5.5d ).
In situ elemental analyses of the skeletally mature normal human or OA human cartilage–bone interface has revealed the presence of calcium, phosphorus, potassium, sulfur, zinc, and strontium.52,53 In mature osteochondral samples, the mineralized surface has a smoother texture and corresponds to the tidemark, with no visible difference by micro-CT between the calcified cartilage and osteoid immediately below ( Fig. 5.5a, b ).54 In a normal human patella, mineralized cartilage showed a slightly but significantly higher calcium content than adjacent bone (25% vs. 23% w/v), and the mineral particles in bone and articular calcified cartilage were found to align with the direction of collagen organization.55 Using two-dimensional nuclear magnetic resonance (2D-NMR) spectroscopy and X-ray diffraction, Duer et al56 analyzed the mineral component of pulverized calcified cartilage samples from skeletally mature horse phalanx and distal radius. They concluded that the calcified cartilage mineral signal is similar to hydroxylapatite of bone, but with smaller peaks indicating small crystals or disorder in the mineral component. They also found evidence that, unlike bone, GAG present in calcified cartilage provides a more hydrated matrix, anionic side-chains (carboxylate and sulfate) for binding calcium in the mineral crystal surfaces, and hydroxyl groups to H-bond with surface water, mineral hydroxyl, and phosphate ions.56 The spectra were also consistent with the presence of Gla residues (γ-carboxyglutamic acid) in the calcified cartilage layer; Gla-domain proteins are found in a variety of mineral-binding proteins such as osteocalcin.27
It is not well understood how the tide-mark is formed, and knowledge of its precise composition is also limited. The tidemark is a 5-µm-thick structure that appears at the cartilage–calcified cartilage junction and can be visualized with hematoxylin, a blue dye that is intensified by metal ions. The tidemark could potentially arise simply by the accumulation and precipitation of chondrocyte-derived extracellular matrix species and ions at the calcified cartilage front, due to the sharp decrease in tissue permeability. The tidemark could serve to inhibit calcifying matrix vesicles released from the bone from penetrating into hyaline cartilage. Lectin staining has suggested that the tidemark contains variously branched alkali-resistant glycans with -galactosyl or N-acetyl-lactosamine termini.57 Zoeger et al53 detected a specific accumulation of lead at the tidemark in normal cadaveric femoral head and patella. Oegema et al suggested that the superficial articular layer could be involved in a paracrine loop that controls deep zone chondrocyte hypertrophy and calcification, which could potentially explain thickening of the calcified cartilage in OA following loss of the superficial zone.26 Alternatively, microcracks in the calcified layer could permit diffusion of bone-derived matrix vesicles farther into the deep zone, resulting in tidemark advancement.
Duplication of the tidemark in aging and OA is well documented.26,44,51,58 In aging subjects, up to 5 duplicated tidemarks were observed in normal human subjects over 70 years old,44 and as many as 10 tidemarks in primates over 20 years old.58 A significant correlation was observed between increasing tidemark duplication, mineral density, and carbonate content in primates.58 Repetitive knee microtrauma in a rabbit model during 9 weeks of loading was shown to lead to a mean 25% increase in the proximal tibial calcified cartilage layer thickness and tidemark duplication, with no change in mean articular cartilage thickness.59 Multiple tidemarks were observed to form at the cartilage–bone interface in tissues surrounding an osteochondral defect in rabbit trochlea 6 months postoperative,60 and in sheep above a metal implant placed in the subchondral bone.59 Tidemark duplication could be related to uneven load-sharing following softening of a focal area of damaged cartilage.60
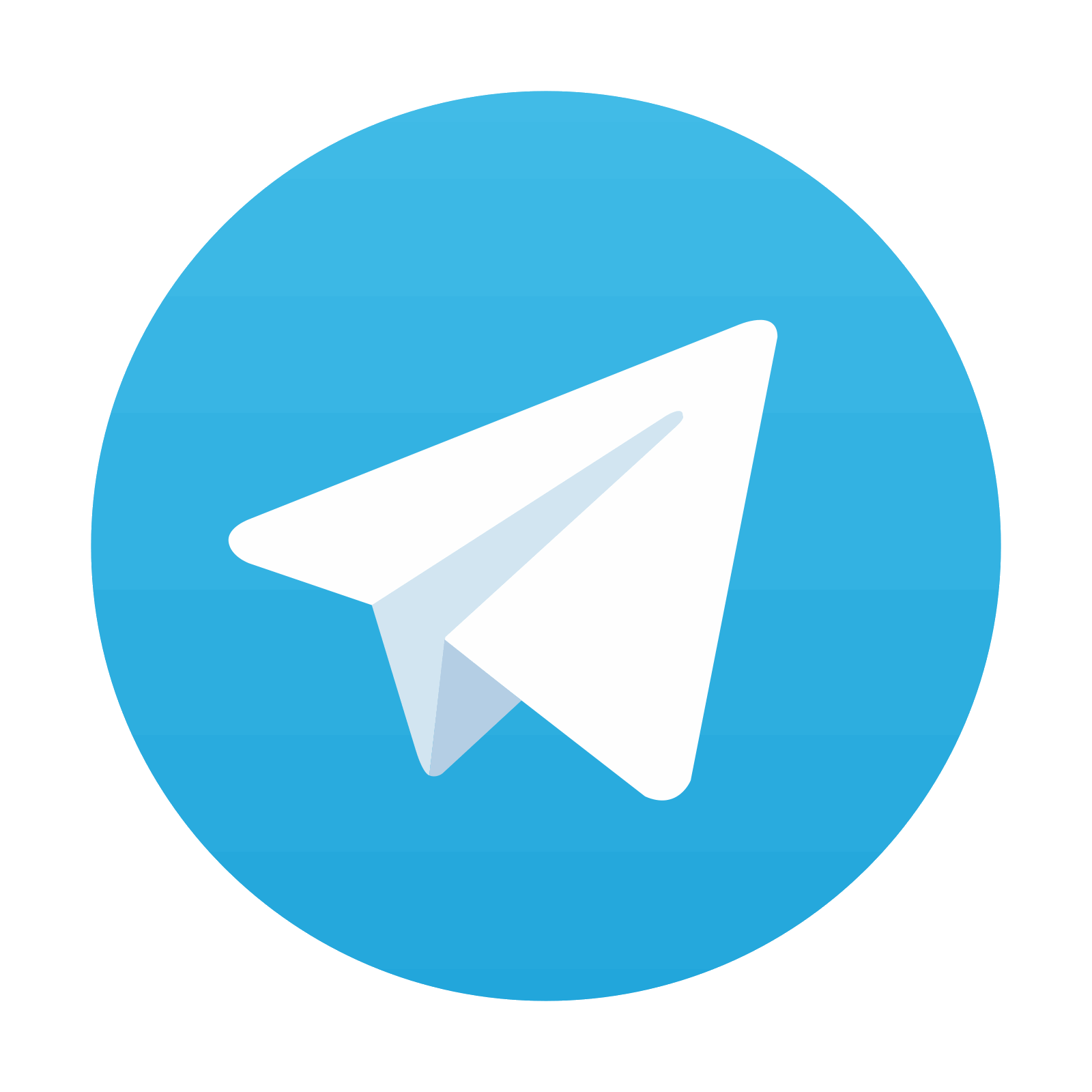
Stay updated, free articles. Join our Telegram channel
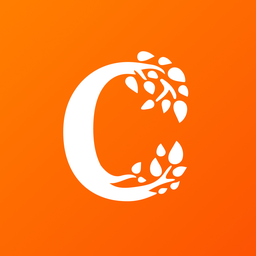
Full access? Get Clinical Tree
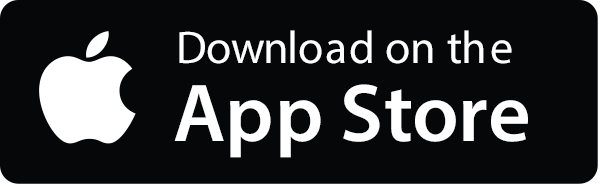
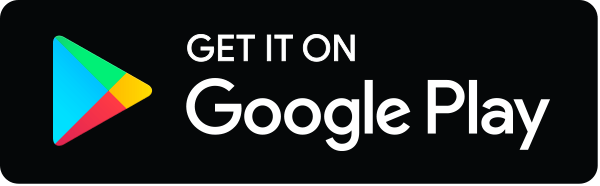