Tendons and Ligaments
Matthew M. Counihan, MD
Louis J. Soslowsky, PhD
Dr. Counihan or an immediate family member serves as a paid consultant to or is an employee of Jazz Pharmaceuticals, Sun Pharmaceuticals, CSL Behring, Biomotive and has stock or stock options held in Merck. Dr. Soslowsky or an immediate family member has received research or institutional support from Embody, Marine Polymer Technology, and Orthofix, Inc.
This chapter is adapted from Reuther KE, Gray CF, Soslowsky LJ: Form and function of tendon and ligament, in O’Keefe RJ, Jacobs JJ, Chu CR, Einhorn TA, eds: Orthopaedic Basic Science, ed 4. Rosemont, IL, American Academy of Orthopaedic Surgeons, 2013, pp 213-228.
Keywords: ligament anatomy and functional properties; tendon anatomy and functional properties; tendon and ligament injuries; tendon and ligament repair and reconstruction
INTRODUCTION
Tendons and ligaments are complex connective tissues and essential components of the musculoskeletal system, aiding in locomotion and assisting in both dynamic and static joint stabilization. The burden of musculoskeletal injuries in the United States continues to expand. The proportion of the population reporting these injuries increased from 76.0 million from 1996 to 1998 to 102.5 million from 2009 to 2011, with a disproportionate amount of this increase occurring among those aged 45 to 64 years. The aggregate economic impact of these injuries from 2009 to 2011 was estimated at $796.3 billion.1 Because tendon and ligament disorders are especially common and are significant contributors to this musculoskeletal injury burden, a clear understanding of their function in health, as well as their dysfunction in pathology and treatment mechanisms, is essential.
Tendon and ligament are frequently discussed together because of their many similarities they share and despite their significant differences. Both have elastic and viscoelastic properties that contribute to their ability to resist a variety of loading scenarios. These behaviors are a result of their complex composition and structural characteristics at each level of organization that lead to their response to joint function.
This chapter will provide an overview of the preclinical concepts behind clinical conditions involving tendon and ligament, highlighting anatomic considerations; gross morphology, microanatomy, and biology; their biomechanical function in the body; relevant injuries; considerations in tendon and ligament repair; and current concepts in tissue engineering for tendon and ligament injuries.
TENDON
CLASSIFICATION AND ANATOMY
Tendons can be classified through multiple schema, according to anatomic considerations: specifically, shape (round or flat), anatomic location (intra-articular or extra-articular; intra-articular soft-tissue injuries have a lower likelihood of healing),2 or the surrounding layer of connective tissue (paratenon or synovium, which acts to reduce friction and allows for tendon gliding).
GROSS MORPHOLOGY, HISTOLOGY, MICROANATOMY, AND CELL BIOLOGY
The connection of the tendon between muscle and its point of origin or insertion at the bone requires several complex anatomic and morphologic characteristics that are continued through the microscopic and molecular level. Primarily, this
connection allows for the transfer of force from muscle to bone and enables highly specific motion by the musculoskeletal system. Not all muscles have a tendon; those that do primarily provide motion at a joint or need to provide force either over a distance or in a tightly confined space. Tendons provide a mechanical advantage for muscle by focusing or redirecting force, lengthening a lever arm, or acting around a pulley (such as in the flexor hallucis longus tendon).
connection allows for the transfer of force from muscle to bone and enables highly specific motion by the musculoskeletal system. Not all muscles have a tendon; those that do primarily provide motion at a joint or need to provide force either over a distance or in a tightly confined space. Tendons provide a mechanical advantage for muscle by focusing or redirecting force, lengthening a lever arm, or acting around a pulley (such as in the flexor hallucis longus tendon).
The round or flat shape of the tendon is associated with specific functional consequences. Classic examples of round tendons include the flexor digitorum tendons of both the upper and lower extremities; flat tendons include the rotator cuff tendons and the Achilles tendon. Most round tendons behave similarly and are biomechanically subjected to a consistent environment. They are accustomed to high tensile loads and are capable of a high degree of motion, or gliding. The flat tendons are less easily categorized. They are generally subject to less glide but are capable of bearing high tensile loads (as in the quadriceps tendon) in addition to more complex loads such as compression and shear. Consequently, these different functions are represented in the structure of these tendons.
Further investigation of tendons reveals a complex composition with more functional implications. At the most basic level, tendon composition is organized consistently throughout the body, despite its often-differing superstructure. Like most connective tissue, the main functional components of tendon are water and collagen. Water is the primary constituent, comprising approximately 50% to 60% of tendon weight. Collagen makes up approximately 75% of the dry weight with 95% type I collagen and a small amount of type III and other collagens. Tendon is also composed of tendon cells, referred to as tenocytes, and proteoglycans. Proteoglycans are composed of a core protein covalently bound to one or more glycosaminoglycan (GAG) chains. GAGs are thought to play an important role in mechanics, specifically viscoelastic properties, through their association with water. The major subcategory of proteoglycans in tendon is the small leucine-rich proteoglycans (SLRPs). The most abundant SLRPs in tendon are decorin and biclycan, also known as class I SLRPs. Other SLRPs, though not as common, are also well described, such as fibromudulin and lumican (class II). Both classes have been found to play significant roles in tendon growth, regulation, and homeostasis. These molecules have been shown to bind to collagen and have significant impact on the hierarchical organization of tendon and its mechanical properties.3 Several investigators have suggested a role for these proteins in tendinopathy, although the specific mechanisms of this relation remain unclear.
At the molecular level, tendons are arranged in a hierarchy starting with the triple helix of collagen, then microfibrils, fibrils, fascicles, and ultimately the tendon itself (Figure 1). The mediated assembly and cross-linking of collagen into these microfibrils is critical for the mechanical strength of the tissue. This process is largely dependent on the family of extracellular SLRPs, which can dictate the overall thickness and quality of fibril assembly.4 Another important element of collagen assembly and structure is crimp. Crimp is evident ultrastructurally and appears as “crimped” lines in the tendon when viewed at the microscopic level. This crimping results from the specific orientation of the microfibrils in the tendon and contributes important mechanical properties that are discussed later in this chapter.
The superstructure of tendons is the progressive organization of fibrils into larger fascicles that group to yield a functional tendon, as well as supporting connective tissue that preserves local anatomic and physiologic relationships. These groups of fascicles are separated and bound together by a thin connective tissue layer known as the endotenon. Contiguous with the endotenon is the epitenon, which envelops the entire tendon. Some tendons are then wrapped with a paratenon
(eg, Achilles tendon), and some are covered with synovium (eg, flexor tendons), depending on the degree of gliding required. The endotenon and epitenon serve to permeate the tendon with vasculature, lymphatics, and nerves.
(eg, Achilles tendon), and some are covered with synovium (eg, flexor tendons), depending on the degree of gliding required. The endotenon and epitenon serve to permeate the tendon with vasculature, lymphatics, and nerves.
Another aspect of tendon’s morphology and functional relationships are its origin and insertion (its junctions). As the tissue that bridges muscle to bone, tendon has two junction regions: the myotendinous junction, where the muscle transitions to tendon, and the bone-tendon junction, where the tendon transitions to bone. Each is differentially organized and contributes to the varying properties along the length of the muscle-tendon-bone unit. The myotendinous junction is composed of the interdigitations of end sarcomeres and connective tissue elements. An end portion of the sarcomere, known as the z-line, which is arranged in a staggered organization with the next sarcomere, splits and gives rise to myofilament bundles that insert directly onto collagen fibrils. This arrangement minimizes stress to the myotendinous junction with forceful contractions.
At the bone-tendon junction, known as the enthesis, there are two typical arrangements with different biomechanical roles: direct or indirect insertions. Direct, or fibrocartilaginous, insertions have been classically characterized by a four-layer transition from tendon to bone: tendon, fibrocartilage, mineralized fibrocartilage, and bone (Figure 2). These insertions are typical in regions where the tendon-bone unit is subject to high tensile loads (such as the rotator cuff).5,6 In an indirect insertion, which is less common than the direct insertion, the tendon fibers insert directly into the periosteum. The fibers that interdigitate with the periosteal tissue have commonly been referred to as Sharpey fibers. These transitions are more common in areas where tensile loading at the tendinous insertion does not predominate (such as the distal head of the rectus femoris).
At the cellular level there are also considerations that have implications for structure and function. Tendon is often classified as relatively hypocellular, yet recent evidence connects tenocytes with a highly defined role in maintenance of tendon structure.7 Tenocytes maintain the extracellular milieu that allows the normal function of tendon by secreting the extracellular matrix, synthesizing collagen and the proteoglycans. They possess long cellular processes that envelop and interdigitate with the collagen fibrils and other tenocyte processes and cell bodies. They are responsive to mechanical loading and can communicate with adjacent tenocytes through gap junctions at their interface as well as by production of inflammatory mediators under stress.7
BLOOD SUPPLY AND INNERVATION
Although tendon is considered largely hypovascular, blood flow has important consequences for tendon in health and disease. The blood supply and innervation of tendon, as mentioned previously, is primarily through the endotenon and epitenon. The tendon is fed primarily through vasculature that enters through the paratenon or at the bony insertions and continues to travel throughout the endotenon. The situation is more complex in sheathed tendons, in which blood supply must enter the tendon through mesotenon in vincula, defined as redundancies of the synovium that tether the tendon to its sheath in certain locations, analogous to the mesentery of the peritoneum (Figure 3). Sheathed tendon is also thought to receive some nutrition through diffusion via the synovial fluid. In addition to tendon’s hypovascularity, there are specific regions of relative avascularity between vascular zones, which are thought to be at particular risk for rupture. These hypovascular areas have been identified in the supraspinatus tendon, long head of the biceps tendon, Achilles tendon, and patellar tendon, all of which are prone to rupture.8
Innervation in tendon includes several types of nerve endings with different functions. Tendon is typically innervated by the same nerve as its muscle. The various special organs include Golgi organs, which provide steady-state information when undergoing large stimulations for prolonged periods; Pacinian corpuscles, which are considered sensitive, fast-adapting mechanoreceptors; and Ruffini endings, which are also very sensitive but can relay information for prolonged periods similar to Golgi organs. These end-organs are typically localized to the myotendinous region. Free nerve endings, responsible for nociception, on the other hand, tend to be clustered at the enthesis.
TENDON FUNCTIONS
Tendon serves the primary purpose of allowing the transmission of force from muscle to bone, providing the capacity for locomotion, as well as allowing dynamic joint stability in concert with ligament, which provides more static restraint to joint translation. This is accomplished through an intricately designed and executed structure that enables these certain biomechanical properties. The relationship between structure and function is paramount.
MECHANICAL
The mechanical function of tendon is to orchestrate a fine balance between mobility and stability. The most obvious aspect of this function is the transmission of muscle-derived force to bone, which provides volitional control of the skeleton. Tendon also possesses the ability to elastically store and transfer energy. This dual ability has protective implications for the musculoskeletal system, as tendon can help absorb force and protect surrounding tissue. The composition of tendon as primarily water and collagen enables it to function viscoelastically, with its stiffness changing in response to loading. This viscoelastic behavior will be discussed later in the chapter.
Another important mechanical function of tendon is to provide secondary restraint to joint motion and add stability to the musculoskeletal system. The insertion of a tendon just distal to the joint on which it primarily acts enables the tendon to buttress against translation of the articular surfaces. A classic example of this function is the hamstring mechanism as a secondary stabilizer of the knee, protecting against anterior tibial translation in the setting of anterior cruciate ligament (ACL) deficiency.
These functional properties of tendon are derived from material and structural properties of the constituents and intrinsic tendon properties as well as the characteristics of the muscle-tendon-bone interface. The intrinsic tendon properties are referred to as material properties because they describe characteristics that are specific to the tendon material itself. This differentiates material properties from the structural properties, which are not normalized by tendon area or length, and thus describe qualities of the whole structure.
Three essential components of tendon function, and both its material and structural properties, are its nonlinear anisotropy and its elastic and viscoelastic behavior. The term nonlinear anisotropy means that tendon mechanical properties differ depending on the direction of applied force and have different values depending on the degree of force applied. Viscoelasticity describes a propensity of many biologic tissues, in which a tissue’s mechanical properties are different depending on the time and recent history of loading the tissue.
Nonlinear anisotropic properties that are important when discussing tendon can be derived from two experimental scenarios. The first, representing structural properties, is called a load-elongation study. In this setting, the tendon-bone unit is elongated while the resultant load developed in the construct is measured (Figure 4, A). At low loads, the tendon is stretched easily (low slope) with nonlinear behavior; this is known as the toe region. This stretching has been attributed to the recruitment and realignment of crimped collagen fibrils (Figure 5). Following the toe region, there is a linear region, where rate of load development is constant. Finally, there is a failure region, at which point load drops off, and stretching is easily accomplished. From this test, the following properties can be derived: stiffness, represented by the slope of the curve in the linear region; ultimate load, represented by the load value at failure; and energy absorbed to failure, represented
by the area under the curve. These parameters are useful in understanding the overall performance of the enthesis in a given experimental setting.
by the area under the curve. These parameters are useful in understanding the overall performance of the enthesis in a given experimental setting.
A more refined measure of the tendon material properties is obtained by the normalization of these structural properties to tendon area and length. This analysis yields a curve known as a stress-strain curve (Figure 4, B). Strain, which represents change in length of the tendon relative to total tendon length, is derived in several ways, the discussion of which is beyond the scope of this chapter. This curve is also nonlinear and represents uniaxial tensile loading of the tendon per area. Several parameters can be determined, including modulus, which is the slope of the curve; tensile strength, which is the stress value at failure; ultimate strain; and strain energy density, represented by the area under the curve.
Both structural and material properties are important in experimental evaluation of tendon and its enthesis, especially when considering tendon repair and healing. It should be recognized that an increase in stiffness alone might not be a positive indicator of repair strength; if there has been a substantial increase in cross-sectional area of the tendon, stiffness may increase while modulus decreases. This is a scenario indicative of an increase in quantity, not quality, of tendon tissue and may not represent successful reconstitution of tendon properties.
VISCOELASTIC
The viscoelastic properties of tendon are essential to understanding how tendon structure contributes to its function. Viscoelasticity can be defined as mechanical behavior that is time- and history-dependent, with material classified by both viscous properties (resisting deformation under stress) and elastic properties (indicating a tendency to resume its original shape when stress is removed). Stated differently, a viscoelastic material subjected to stress will undergo an initial deformation, but the same stress will cause less deformation with time. Tendon behavior tends to be dominated by viscous properties at low loads and more elastic behavior at high loads.
Viscoelastic behavior can be characterized by creep, stress relaxation, hysteresis, and strain rate sensitivity parameters (Figure 4, C). Under a constant applied load, tendon will undergo initial deformation and then continue to elongate at a much slower rate, without any further increase in applied load. This tendency is known as creep. Another aspect of this phenomenon can be seen in the case of a constant elongation. In this scenario, the elongation is the independent variable, held constant. The load in the tendon is the measured, dependent variable. With time, the load will decrease. This behavior is known as stress relaxation. Tendon also displays a behavior responsive to its loading history. If tendon is cyclically elongated to a set length and allowed to relax, and the load is measured, the tendon will unload along a different curve than in loading. The area between the two curves represents dissipated energy and is known as hysteresis. Furthermore, repeated cycling will give rise to progressively smaller loads in the tissue, consistent with stress relaxation, in a pattern known as cyclic load relaxation. In addition, when a repeated stress is applied, nonlinear changes occur in the deformation, resulting in cyclic creep.
PROPRIOCEPTION
Tendon has been shown to have excellent innervation for the purpose of proprioception, or the sensing of joint position in space. This ability enables tendon to contribute more effectively to biomechanical function. Clinically, an appreciation of proprioception is relevant because of tendon repair and transfer surgeries. If proprioception is not reestablished, tendon repair outcomes seem to be diminished. As an example, the outcome of teres major transfer to the supraspinatus insertion for massive rotator cuff tear is dramatically improved if the teres can be retrained to function as an arm abductor, which requires preservation of proprioception in the teres tendon.9
BIOLOGIC FACTORS INFLUENCING TENDON PROPERTIES
Several biologic factors have significant effects on tendon properties, including age, presence of comorbidities, activity level, and anatomic location. Specifically, studies have shown that tendons from older individuals are prone to increased incidence of tendon injury.10,11 Additionally, medical comorbidities can lead to in vivo degradation of tendon; diabetes, hypercholesterolemia, and kidney disease each have been identified. Exercise can also profoundly affect tendon, with exercised tendons showing increased modulus compared with age-matched control tendons.12 Anatomic location also significantly affects tendon tensile strength. As shown previously, tendon from the erector spinae muscles have modulus values an order of magnitude higher than the rotator cuff tendons.13 These factors apply to most connective tissue and will be discussed in more detail in the Ligament section.
EXPERIMENTAL FACTORS INFLUENCING TENDON PROPERTIES
Several technical aspects must be considered when performing experimental measurements as a method to determine tendon mechanical properties. These include the appropriate testing environment, specimen type and orientation, strain rate, and specimen storage, as well as biologic conditions; samples must be similar in age at the moment of harvest and these must be similar in size and diameter.
Testing Environment
The environment for testing has a significant effect on mechanical properties. Specifically, the hydration and temperature of the tissue should be taken into consideration. For example, studies have shown that tendon stiffness decreases with increased temperature.13
Specimen Type and Orientation
As described previously, tendon is composed of a bone-tendon-muscle complex. To examine the structural properties,
the entire complex is often tested experimentally. The specimen is typically positioned in an anatomic orientation, so that the amount of fibers engaged along the longitudinal axis during loading is maximized. In addition, to examine material properties, the attachments to the tendon are often removed and the tendon is tested independently of the bone. In each of these situations, the specimens must be gripped differently to determine tensile properties. To minimize the error as a result of slipping and stress concentrations at the grips, the tendon should have a high length versus width ratio, defined as an aspect ratio.
the entire complex is often tested experimentally. The specimen is typically positioned in an anatomic orientation, so that the amount of fibers engaged along the longitudinal axis during loading is maximized. In addition, to examine material properties, the attachments to the tendon are often removed and the tendon is tested independently of the bone. In each of these situations, the specimens must be gripped differently to determine tensile properties. To minimize the error as a result of slipping and stress concentrations at the grips, the tendon should have a high length versus width ratio, defined as an aspect ratio.
Finally, the rate of loading applied experimentally and proper specimen storage also have significant effects on tendon properties. These factors are characteristic of most soft tissues and will be discussed in more detail in the Ligament section.
CLINICALLY RELEVANT INJURIES
Tendon injuries are an important aspect of musculoskeletal medical conditions. There are several types of tendon injury, ranging from overuse injuries (such as tendinosis) to rupture, with different clinical consequences. Tendon ruptures are classified into direct and indirect ruptures based on mechanism: direct injuries involve direct trauma to the tendon causing a tear, whereas indirect injuries result from forceful loading of the muscle-tendon-bone unit to the point of tendon failure.
Tendinopathy is an umbrella term for a group of syndromes on the same spectrum, considered to be overuse injuries, resulting in pain and varying degrees of loss of function of the tendon. Tendinosis has largely replaced the term tendinitis in the orthopaedic literature to describe the chronic overuse injury to tendon, as true inflammation is generally absent in these patients. These injuries are characterized by persistent pain and radiographic evidence of intrasubstance tendon degeneration.14 Upon histologic examination, the hallmarks of tendinosis include disrupted and disorganized collagen, increased extracellular matrix, increased tenocyte population, and neovascularization. Tendinosis, in addition to being a clinical problem itself, can lead to more severe injury, such as complete tendon rupture. Direct and indirect ruptures of tendons remain an important orthopaedic problem, with ongoing management challenges. Injury to the flexor tendons of the upper extremity is the most common and highly characterized of the direct tendon injuries. Penetrating trauma is usually responsible, with stab wounds making up a large portion of these injuries. These tendons typically have no preexisting condition that predisposes them to injury. The tendon is lacerated and retracts because of its muscular attachment. Additionally, the tendon sheath is violated, introducing the risk of infection into a hypovascular space and depriving the tendon of the normal synovial fluid, through which it obtains a portion of its nutrition.15
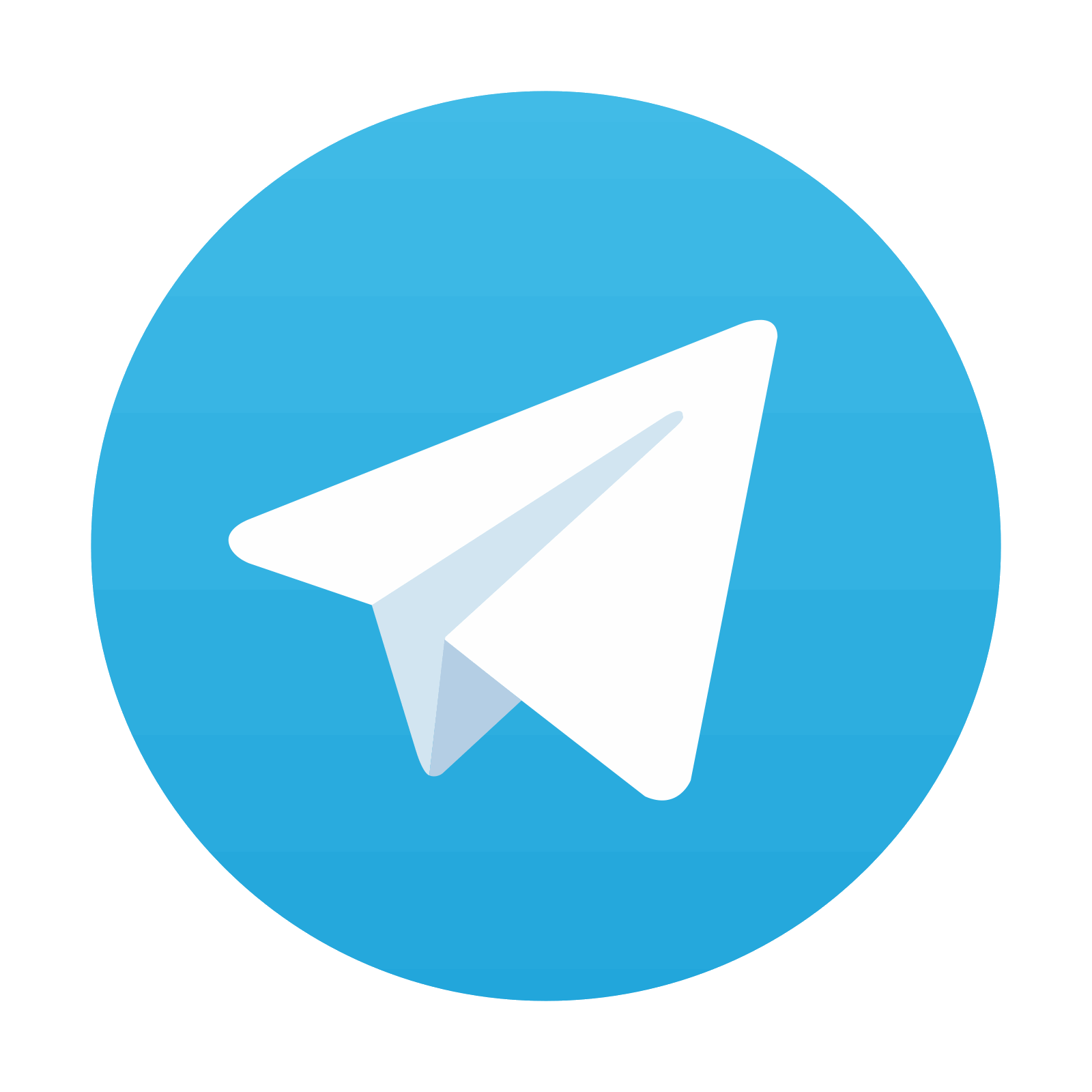
Stay updated, free articles. Join our Telegram channel
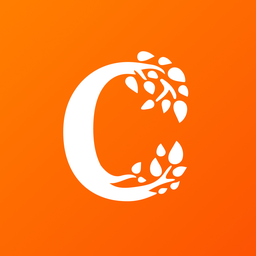
Full access? Get Clinical Tree
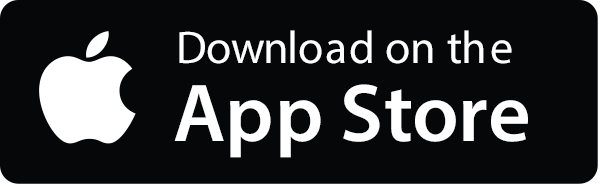
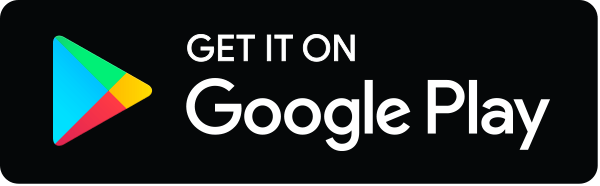