Fig. 22.1
Targeted muscle reinnervation concepts. (a) In an uninjured limb, the cortical system serves as the control center and initiates voluntary movement. Motor nerves function as an electrical conduit and transmit the signal to a target effector (muscle). Contraction of the muscle results in movement across a particular joint. (b) After amputation, there is variable loss of muscle effectors and terminal motor nerves. However, the control center and proximal ends of the electrical conduits remain intact and functional

Fig. 22.2
Targeted muscle reinnervation schematic. (a) In the setting of amputation, distal motor nerves are variably injured, innervate absent or dysfunctional muscle effectors, and/or are prone to painful neuroma formation. (b) With targeted muscle reinnervation, select motor nerves of the brachial plexus are rerouted to new functional muscle effectors. By surgically redirecting these nerves, independent electromyogram signals can be generated via voluntary contractions by the patient. Skin surface electrodes then detect these signals and initiate myoelectric prosthesis function, allowing for more intuitive control of advanced robotic arms
By reorganizing the orientation of terminal branches of the brachial plexus, a greater number of myoelectric control signals can be distinctly activated to mimic the original functions of those nerve/muscle units. The musculocutaneous, median, ulnar, and radial nerves are all present at a transhumeral amputation site and could theoretically generate unique EMG signals to control different prosthetic motors. The musculocutaneous nerve normally controls elbow flexion via the biceps brachii, brachialis, and coracobrachialis. Redirection of the musculocutaneous nerve to generate a surface EMG signal could be used to activate prosthetic elbow flexion, similar to its native function. Likewise, a rerouted radial nerve could activate prosthetic elbow or hand extension via surface EMG signals, similar to its original role as an extension signal in an intact limb.
Animal and human studies have demonstrated that in newly created nerve motor units (e.g., cross-innervated muscle), motor control is dominated by the original function of the nerve, not the function of the muscle [11–13]. By exploiting the nerve’s intrinsic motor programming, the prosthetic control mechanisms can be made more intuitive and natural which ultimately translates into greater function. Importantly, functional muscle–nerve units are left intact during TMR procedures, and only residual nerves are used to minimize additional morbidity. It is also critical to preserve shoulder biomechanics so that body-powered functions are not lost.
For TMR to be successful, three requirements have been described [11]. First, multiple nerves need to adequately reinnervate distinct areas of the muscle and skin consistently. Second, independent signals with minimal surrounding interference (i.e., muscle cross talk) must be detected from each myoelectric control area (i.e., patch of the skin). Third, a prosthesis must be able to receive myriad EMG inputs, integrate multiple motor functions, and provide sensory feedback. Early experience with TMR highlights the feasibility and potential of this treatment paradigm for proximal upper limb amputees [14].
Integration with Bioprostheses
Modern prostheses can be “cosmetic” and appear quite similar to a normal upper limb at the expense of minimal function. Conversely, “functional” prostheses aim to restore upper limb movements using body power (e.g., shoulder movement), external (e.g., electric) power, or both in a “hybrid” approach. Body-powered prostheses use harnesses and cables to convert shoulder motion to prosthetic function and rely on patient strength. Only one component of the prosthesis can be activated at a time and must be locked into place before a different component can be activated, resulting in “sequential” control.
External prostheses are generally based on electric power and use motors to create movement. Most are myoelectric, i.e., they use EMG signals from terminal muscles at the amputated limb to control motorized components. Complex movements are limited based on the number of independent control signals. Similar to body-powered systems, only sequential control is able to operate separate prosthetic functions. Ideally, antagonistic flexion and extension muscle pairs would be used to control a joint with independent feedback mechanisms and minimal adjacent muscle cross talk. This approach is more useful for distal amputations such as transradial but difficult with high-level proximal amputations because there are fewer physiologically appropriate muscle groups.
TMR has been shown to improve control and function of myoelectric prostheses in validated functional tests [14]. Patients demonstrated markedly improved gross hand function in block movement and clothespin relocation tests. Patients also reported subjective improvements in hand control and lesser disability after TMR surgery. However, the nerves transferred during TMR transmit signals far more complex than just an “on–off” cue to a single muscle [15]. Conventional myoelectric systems can only receive defined EMG amplitudes at specific control sites, thus failing to incorporate more nuanced information needed to perform more complex tasks.
One approach to better capture this neural information is via pattern recognition algorithms that utilize machine learning to predict intended movements [11, 16]. TMR patients provide sample contractions for each of their myoelectric prosthesis movements. A computer algorithm then “learns” the EMG patterns and can facilitate future motions over time, allowing for improved coordination and greater complexity of movement. EMG patterns, including more than just signal amplitude, are detected over both native and reinnervated muscles to capture patterns in regional activity with each motion.
Myoelectric control systems are largely limited by the difficulty in detecting adequate EMG signals and the scarcity of information needed to control multiple functions. To address these challenges, new technologies are being developed to improve the nerve–prosthesis interfaces [7]. Implantable electrodes have been designed to record myoelectric signals directly from the muscle, thus obviating the need for skin surface conduction which is affected by environmental factors [17]. Implantable devices would need to be powered for long-term recording and be compatible with telemetry systems that function in deeper tissues.
Direct access to peripheral nerves is another strategy to improve the resolution of cortical signals. However, these signals are almost a thousand times smaller than EMG signals, thus requiring sensitive electrodes for recording and stimulation [7]. Electrodes can be extraneural or intraneural, the latter demonstrating greater precision and sensitivity. Current limitations include the risk of nerve damage with intraneural electrodes and inconsistent results, because only a fraction of a nerve’s individual action potentials can be captured by a single electrode.
Researchers have also explored capturing electric potentials directly from the brain. Neuronal activity can be recorded from inside, on the cortex surface (electrocorticogram), or over the scalp (electroencephalogram) [18]. The motor and somatosensory cortices contain a topographical map of the human body and thus represent a potential interface to detect neuronal activity. Research in these areas is actively ongoing but requires significantly more investigation before widespread clinical testing and adoption.
Surgical Considerations
Successful application of TMR is based on an understanding of brachial plexus and upper extremity peripheral nerve anatomy. Briefly, it is a plexus of somatic nerves formed by the ventral roots of the lower four cervical nerves (C5–C8) and the first thoracic nerve (T1). It innervates all muscles of the upper extremity except for the trapezius and levator scapula. The brachial plexus is subdivided into roots, trunks, divisions, cords, and branches. The major terminal branches innervate muscle groups with distinct functions and include the median, ulnar, musculocutaneous, and radial nerves. By rerouting these motor nerves to defined muscle skin areas, surface electrodes can detect independent signals that activate prosthetic functions aligned with the “original” nerve function.
Another important anatomic concept underlying TMR is agonist–antagonist muscle groups. For arm movement, nerve activation of a muscle agonist (e.g., the biceps brachii for elbow flexion) requires inactivation of the corresponding muscle antagonist (e.g., the triceps brachii for elbow extension). For example, in an intact arm, elbow flexion would be activated by musculocutaneous nerve stimulation of the biceps brachii, whereas radial nerve stimulation of the triceps brachii would be absent. During TMR surgery, the musculocutaneous and radial nerves would be rerouted to distinct areas so that they could activate opposing movements of the prosthesis, similar to a normal arm.
As part of the process in evaluating patients for TMR, reconstructive surgeons assess the amputation site for evidence of adequate healing and durable soft tissue coverage. Certain areas of the limb may have an excess of subcutaneous fat or scar that may dampen the myoelectric signal at the skin surface. This may require subcutaneous thinning procedures or excision of the scar tissue. Surgeons have developed techniques to place flaps of adipose tissue between adjacent muscle segments to isolate myoelectric signals and minimize muscle cross talk [19]. Areas of heterotopic ossification (ectopic bone formation in the soft tissues) may be present from the initial injury and may interfere with prosthetic fitting. Skin graft coverage may have been used initially for reconstruction but may not be ideal for long-term prosthetic wear. Pedicled flaps or free tissue transfer may help to deliver thick vascularized tissues to the amputation site to establish a more durable stump or prosthetic fitting site. Free muscle flaps can even be innervated to provide functional motor units and new targets for TMR.
The level of upper limb amputation is also critical in surgical planning to facilitate TMR. For elbow disarticulations or long transhumeral amputations, the limb may need to be shortened and/or angulated for prosthesis wear. TMR should be considered at the time of elective amputation or revision so that nerves can be protected and transferred earlier in the recovery process [20]. The following technique has been described by Dumanian and Cheesborough [19, 20]. Two incisions, both dorsal and ventral, are made in the upper arm to identify motor nerves using a nerve stimulator. The median nerve is transferred to the motor nerve of the short head of the biceps brachii to activate prosthetic hand closure. The long head of the biceps brachii remains intact for prosthetic elbow flexion via the native musculocutaneous nerve. Via the dorsal incision, the long head of the triceps brachii remains intact for prosthetic elbow extension via the native radial nerve. The distal radial nerve is transferred to the motor nerve of the lateral head of the triceps brachii to activate prosthetic hand opening. The ulnar motor nerve can also be transferred to the brachialis to provide an additional myoelectric control point.
TMR for shoulder disarticulation patients is extremely challenging for multiple reasons, including the short nerve lengths, the paucity of muscle targets, and the severe soft tissue defects associated with the injury. However, the prosthetic goals are identical to those for more distal level amputations: elbow flexion/extension and hand opening/closing. Trunk muscles available for reinnervation include the pectoralis major and minor, serratus anterior, and latissimus dorsi. Additionally, the pectoralis major may have muscular subdivisions (clavicle, superior sternal head, inferior sternal head) that can be individually reinnervated. Specific techniques have been previously published, but particular emphasis should be placed on transfer of the musculocutaneous nerve for prosthetic elbow control and the median nerve for prosthetic hand closing [20, 21].
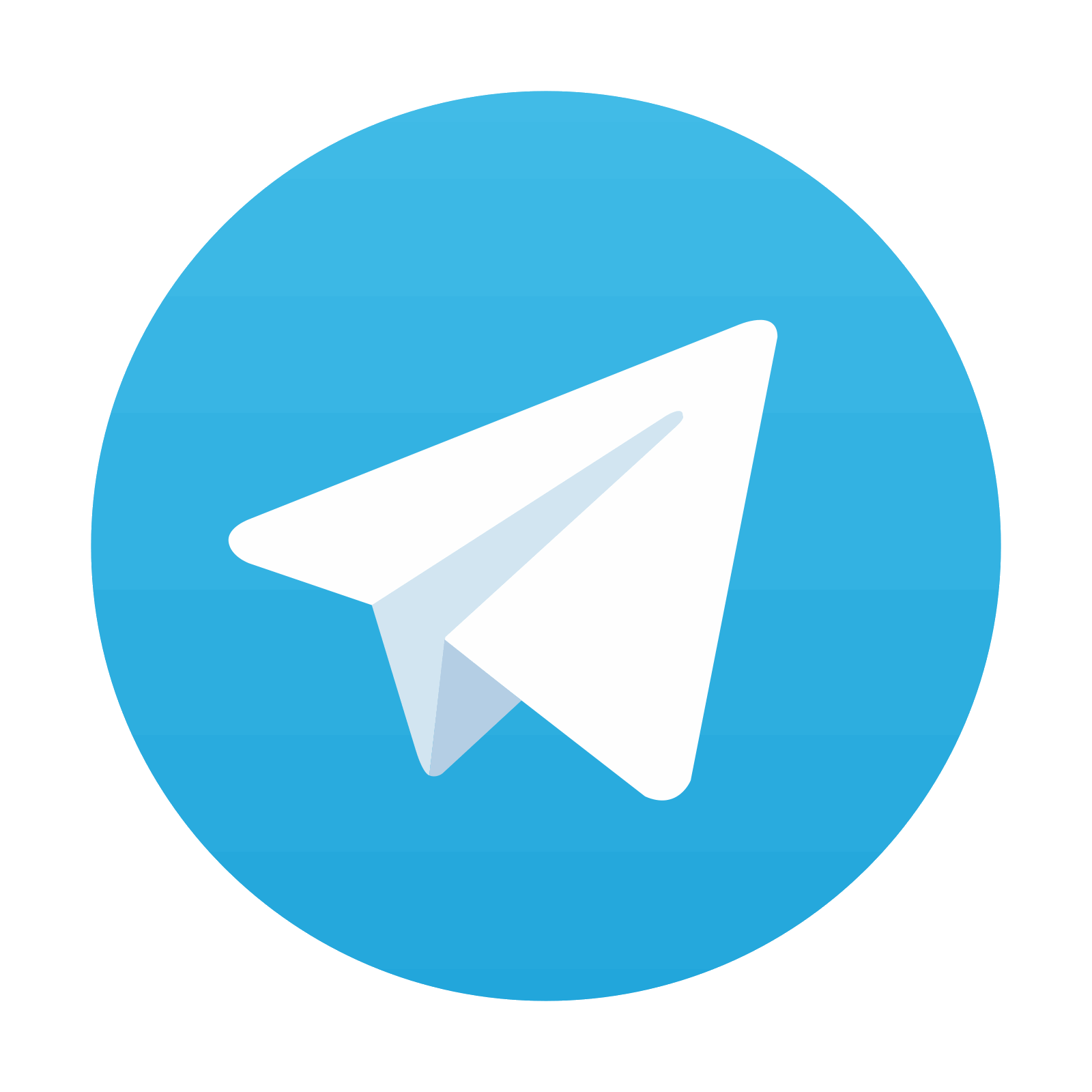
Stay updated, free articles. Join our Telegram channel
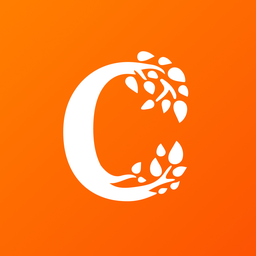
Full access? Get Clinical Tree
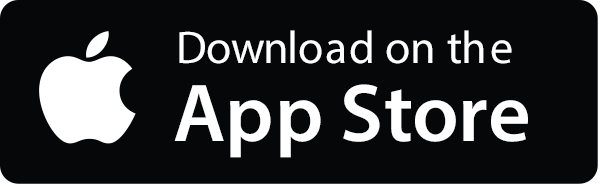
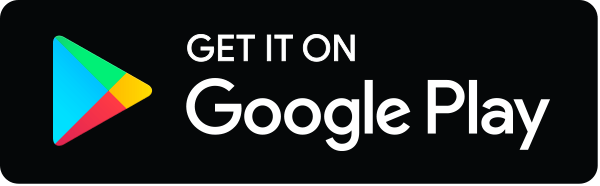