Food or drink
Serving
Caffeine (mg)
Expresso coffee
1 standard serving
107 (25–214)
Iced tea
600 mL
20–40
Chocolate milk
60 g
5–15
Dark chocolate
60 g
10–50
Coca-Cola
330 mL
43
Red Bull energy drink
250 mL
80
PowerBar sports gel
40 g
25
Caffeine, as well as their metabolites (especially theophylline and paraxanthine), is structurally very similar to adenosine and can bind to their receptors on the cell membrane, thereby blocking its action [42]. Adenosine receptors are ubiquitous in the human body, which promotes the action of caffeine in various tissues simultaneously, resulting in a variety of physiological responses that interact with each other [43].
In light of current knowledge, it is not possible to explain precisely the dominant mechanism by which caffeine exerts its ergogenic action. Nevertheless, it is known that caffeine exerts broad physiological effects at central and peripheral level, in particular on the sympathetic nervous system, muscle, endocrine, cardiovascular, pulmonary, and renal levels [44, 45]. The effects of caffeine on the central nervous system possibly include changes on the level of sympathetic activity, perception of fatigue and pain, and recruitment of motor units [43]. Caffeine is known for its effects in enhancing capabilities related to alertness and ability to concentrate, including, but not limited to, sleep deprivation, possibly by stimulating the sympathetic nervous system and/or antagonism of adenosine. In this sense, there is evidence of positive effects of caffeine action in tasks such as surveillance, visual information processing, reaction time, motor learning, humor, and possibly short-term memory [46]. A meta-analysis has shown that caffeine reduces at 5.6% the degree of perceived exertion, compared to placebo, demonstrating clearly that this aspect may partially explain the ergogenic effects of caffeine in sports performance [47]. Although not consensual [48–51], especially in high- or maximum-intensity activities, some authors admit that caffeine can maintain or increase the level of firing of motor units, resulting in greater force produced by the muscle, explaining the positive effects on anaerobic performance [52]. Caffeine also exerts action at peripheral level. Caffeine can increase circulating levels of catecholamines, wherein at least this increase is not the most important parameter for ergogenic action of caffeine [43, 52]. Another mechanism proposed for the ergogenic action of caffeine is to promote the oxidation of fat and inhibiting oxidation of carbohydrates. It has been suggested that this process results in reduced dependence on muscle glycogen reserves [53]. However, although accepting the possibility that caffeine leads to the mobilization of nonesterified fatty acids from adipose tissue, there is no evidence that consequently there is an increase in fat oxidation in the muscle or an increase in muscle glycogen savings [54].
The ergogenic effect of caffeine in team sports (high intensity, intermittent) has been shown in most [55, 56] but not all studies [57]. Considering football [58], although not entirely consensual [59], it was found that caffeine intake led to improved performance through the enhancement of various parameters:
Improvement of cognitive functioning: increased capacity of interpretation and response to visual and auditory stimuli (e.g., react more quickly and accurately to the request of a colleague-like “pass”) [56]
Reduced decline in technical capabilities during a game: increased passing accuracy; improved fine motor qualities such as ball control; improved ability to dribble [56, 60]
Increased countermovement jump height, average peak running speed, total running distance, number of sprint bouts, and running distance covered at a high speed [63]
Higher mean sprint speed [66]
Reduced decline in sprinting performance throughout the game [62]
Increased number of sprints and total distance covered at high intensity [61]
Increased peak power in sprint [67]
Reduced decline in the ability to perform mental tasks toward the end of the game: caffeine attenuated the reduced feelings of energy and increased feelings of fatigue; resulted in more rapid responding, improved target identification, and reductions in false alarms [68]
Altered perception of fatigue and effort that enables players to better maintain voluntary sprinting and jumping ability [62]
Most studies testing caffeine as ergogenic use the administration of dosages on the order of 6-mg/kg body weight [50, 55–57]. However, there is evidence demonstrating the beneficial effects of the ingestion of lower amounts of caffeine (1–3-mg/kg body weight) [46, 70, 71]. There is no dose-response relationship between caffeine intake and the benefits in endurance exercise performance, or if this relationship exists, there is a stabilization to 3-mg/kg body weight [72, 73]. On the other hand, it is known that cytochrome P450, responsible for metabolization of caffeine by the liver, becomes saturated with caffeine intake greater than 5-mg/kg body weight. Accordingly, higher doses entail the risk of producing disproportionate increases in plasma concentration of caffeine. In football, the optimal dose intake may vary according to the position on the field. The dosage required to exert an optimal effect on the visual processing is substantially lower than that required to exert an optimal effect on endurance capacity. Furthermore, although not consensual [56], it is thought that possibly a too high dose of caffeine can adversely affect processing of visual information [74]. So, considering that a goalkeeper does not need a great endurance capacity [75] and that abilities such as reaction time, alertness, and processing of visual information are crucial to his success, he should ingest a dose of 1–2-mg/kg body weight. On the other hand, a field player should ingest between 3- and 5-mg/kg body weight [74]. Doses in the range of 6–9-mg/kg of body weight can have side effects such as increased anxiety, sleep disturbances at night after an evening match [76, 77], and exaggerated increase in heart rate [78].
Caffeine is rapidly absorbed (about 20 min, in the stomach) and reaches peak concentration in blood plasma in 1 h [43]. Given that caffeine is catabolized slowly (half-life is from 4 to 6 h) and concentration in circulation is maintained near the maximum in 3–4 h following administration [43], caffeine intake 1 h before the game (before warming up) enables an ergogenic effect during the entire game, even if there is extra time [74]. During the game, caffeine mouth rinse (alone or in combination with carbohydrates) may rapidly enhance power production, which could have benefits for specific short sprint exercise performance [67], but not maximum strength or muscular endurance performance [79].
53.3 Carnitine
Fat and carbohydrate are the primary fuel sources for skeletal muscle during a football game [80, 81]. Fat constitutes the largest energy reserve in the body, and, in terms of the amount available, it is not limiting to endurance exercise performance [82]. However, fat exhibits a relatively low maximal rate of oxidation, which begins to decline at around 65% of maximal oxygen consumption (VO2max) when muscle glycogen becomes the major fuel supporting ATP homeostasis [83]. The muscle glycogen stores are limited, and it has been well established that muscle glycogen depletion, or partial depletion, in a significant number of fibers coincides with fatigue in the final phase of a football game [84–86]. As fatigue can be postponed by increasing pre-exercise muscle glycogen content [87], it is thought that augmenting the rate of fat oxidation during endurance exercise could delay glycogen depletion and improve endurance exercise performance.
Carnitine (L-3-hydroxytrimethylamminobutanoate) is a naturally occurring compound that can be synthesized from the essential amino acids lysine and methionine or ingested through diet. The rate of carnitine biosynthesis in humans has been estimated to be about 1–2-μmol/kg body wt/day [88]. Nonvegetarian humans obtain an additional ~1 mg/kg of carnitine per day from dietary sources [89]. Primary sources of carnitine are red meat and dairy products (e.g., steak, 95.7 mg/100 g) [90]. The recommended upper limit of L-carnitine supplementation is 2 g/day [91], but no adverse effects were reported following feeding up to 6 g/day for 1 year [92]. Skeletal muscle contains more than 95% of the total body carnitine (~20 g of carnitine in a 70-kg man, of which more than 19 g is in skeletal muscle), as either free or acylcarnitine [93].
Carnitine plays two essential roles in muscle energy metabolism. In the absence of carnitine, the inner mitochondrial membrane would be impermeable to long-chain fatty acids and fatty acyl-CoA esters [94]. Carnitine and carnitine palmitoyltransferase (CPT) are essential for the translocation of long-chain fatty acids into skeletal muscle mitochondria for β-oxidation. A second broad function of carnitine involves the formation of acylcarnitines from short-chain acyl-CoA. The generation of the acylcarnitine serves to buffer the small, dynamic coenzyme A pool against metabolic transients and protects against acyl-CoA accumulation, which may be deleterious to cellular function [95]. The rationale behind carnitine supplementation is that increasing muscle carnitine content could potentially increase fat oxidation during prolonged exercise, spare glycogen stores, and, thus, delay the onset of fatigue [96].
The majority of the pertinent studies in healthy humans to date have failed to increase skeletal muscle carnitine content via oral or intravenous L-carnitine administration. The bioavailability of orally administered carnitine is ∼16–18% at doses of 1–2 g and may be even lower at higher doses [93]. Carnitine is transported into skeletal muscle against a considerable concentration gradient (>100-fold) which is saturated under normal conditions, and so it is unlikely that simply increasing plasma carnitine availability per se will increase muscle carnitine transport and storage [97]. A 1 g oral dose of carnitine will increment the total body carnitine pool by ∼0.08 g or 0.4% [93]. However, insulin appears to stimulate skeletal muscle carnitine transport, and intravenously infusing L-carnitine in the presence of high circulating insulin (>50 mU/l) can increase muscle carnitine content by 15% [96].
A recent study [98] showed an increase in total muscle carnitine content following 2 g of carnitine plus 80 g of carbohydrate ingestion twice daily for 6 months. There was an 80% increase in free carnitine availability during 30 min of exercise at 50% VO2max compared to control and a 55% reduction in muscle glycogen utilization. The study also demonstrated an improvement in exercise performance in all participants (11%, on average) during a 30-min cycle ergometer time trial in the carnitine-loaded state. Whether these improvements in endurance performance are due to glycogen sparing as a result of a carnitine-mediated increase in fat oxidation or the reported effect of carnitine on glucose disposal requires further investigation, but due to the nature of the time trial, this seems unlikely.
In which concerns to body composition, a recent study showed that a 20% increase in muscle carnitine content prevented the 1.8-kg increase in body fat mass associated with daily ingestion of a high-carbohydrate beverage [99]. Moreover, this maintenance of body mass was associated with a greater whole-body energy expenditure during low-intensity physical activity accounted for by an increase in fat oxidation. In line with the role of carnitine in the translocation of long-chain acyl groups via CPT1, these findings are most likely due to an increase in the rate of fat oxidation compared with control at rest and during low-intensity exercise [99]. Overall, however, the practical implications of this are currently unclear, and there is not enough evidence to recommend carnitine for weight loss or to increase fat oxidation [100].
Published data regarding L-carnitine supplementation on footballers is still scarce. Orer et al. [101] examined the effect of acute L-carnitine loading (3 or 4 g) on the endurance performance of footballers. The authors verified that L-carnitine supplementation taken before physical exercise prolonged time to exhaustion. Naclerio et al. [102] investigated the effects of ingesting a multi-ingredient supplement composed of carbohydrate, whey protein, glutamine, and L-carnitine on intermittent performance, perception of fatigue, immunity, and functional and metabolic markers of recovery in footballers. Although the perception of fatigue and serum myoglobin was attenuated for the multi-ingredient group, the supplement did not improve intermittent performance, inflammatory, or immune function. Finally, Guzel et al. [103] assessed the effect of acute L-carnitine supplementation on nitric oxide production and oxidative stress after exhaustive exercise in young football players. The authors found that L-carnitine supplementation (3-g dose) provides strong antioxidant action by increasing the antioxidant glutathione and nitrate-nitrite level and decreasing the thiobarbituric acid reactive substances (TBARs) level.
53.4 Creatine
Creatine is produced from three amino acids (glycine, arginine, and methionine) endogenously by the liver (1–2 g), or it can be obtained from exogenous sources. By adequate intake, some creatine is also added to the pool, predominantly from meat and fish, with a typical diet supplying approximately 1–2 g of creatine daily [104]. Almost all the creatine in the body is stored in skeletal muscle (95%), around 60% in the phosphorylated form (phosphocreatine). It represents an average creatine pool of about 120–140 g for an average 70-kg person [105].
Phosphocreatine serves as a readily available source of phosphorus in skeletal muscle and other tissues. The fast rephosphorylation of ADP from phosphocreatine, via the creatine kinase reaction, buffers changes in ATP during transitions between rest and exercise and contributes a substantial fraction of ATP synthesis during short-duration, high-intensity exercise. Net phosphocreatine hydrolysis consumes hydrogen ions so it may also contribute to buffering of intracellular acidosis during exercise [106]. Creatine supplementation does not increase skeletal muscle protein synthesis as was thought earlier [107].
The relative importance of phosphocreatine during exercise depends on the nature of the exercise [106]. There is a consensus that exercise performance that involves short periods of extremely powerful activity (1–2 s) can be enhanced by creatine supplementation, especially if the activity is performed in repetitive bouts separated by short rest periods (30 s–1 min) [105]. On the other hand, creatine supplementation does not appear to enhance aerobic-oriented activities [106]. Highly trained athletes who participate in sports in which performance relies on repeated efforts could benefit from creatine ingestion by means of an increased ability to perform intermittent high-intensity exercise bouts [108]. This would be the case in football because high-intensity running is a crucial element of performance. Across seven seasons (2006–2007 until 2012–2013) in the English Premier League, high-intensity running distance and actions increased around 30%, and sprint distance and number of sprints increased around 35% [109]. In this context, creatine supplementation is also of particular interest for football players given that phosphocreatine stores exhibit significant declines during football match play [80].
There is compelling evidence of benefits of creatine supplementation in football. Acute creatine supplementation favorably affected repeated sprint performance and limited the decline in jumping ability [108]. On the other hand, a study with 16 male amateur football players suggests that short-term creatine supplementation does not enhances repeated high-intensity or prolonged football-specific exercise performance and body mass [110]. Creatine supplementation may also be useful during a preseason training preventing the decrement in lower-limb muscle power [111]. Additionally, creatine supplementation may also be beneficial in football players proceeding rehabilitation, with a faster recovery from muscle atrophy [112].
Most studies have employed a “loading” dose of 20 g creatine per day for 4–6 days. A more recent approach suggests that a lower dose of 3 g creatine per day will increase muscle total creatine to the same values; however it takes about 30 days [106]. To maximize creatine storage to a given dose, it is also recommended that creatine be consumed post-exercise and in conjunction with carbohydrate and/or protein feeding given that elevated insulin is known to increase muscle creatine uptake [113].
Few reports from athletes have claimed that creatine supplementation may induce muscle cramps. However, it appears that muscle cramping might be due to the intensity of exercise rather than creatine supplementation itself [104]. Despite published allegations of detrimental effects of oral creatine supplementation on liver metabolism, studies on humans have not shown any significant increase in plasma urea, nor liver enzyme activity, during 5 years of creatine supplementation. No reports have observed a modification of the glomerular filtration rate or the presence of microalbuminuria [104]. Creatine supplementation should not be used by athletes with pre-existing renal disease or those with a potential risk of renal dysfunction (diabetes, hypertension, or reduced glomerular filtration rate) [104].
Acute creatine supplementation can also induce a 1–1.5-kg gain in body mass, an effect that is greater in men compared with women [114]. Such increases in body mass are confined to fat-free mass and are likely due to an increase in intracellular water accumulation. For this reason, not all football players should be supplemented with creatine given the perception that they feel heavier and slower. It is conceivable that this increase in body mass in a weight-supported sport like football could decrease performance by increasing the energy cost of running.
53.5 Omega-3
N-3 fatty acids, a class of polyunsaturated fatty acids (PUFAs) found in various cold-water fish (salmon, mackerel, sardines, and herring), have been implicated in a number of metabolic effects with potential benefit in the sport setting [115]. N-3 fatty acids may be useful in the process of dampening inflammatory responses [116, 117], although its importance in ameliorating post-exercise inflammation is not consensual [118, 119]. The potential implications of reducing post-exercise inflammation would be reduced pain and quicker recovery time from intense exercise. Some data show beneficial effects on delayed onset of muscle soreness after only 7 days of supplementation. This suggests that chronic supplementation is not necessary for protection against muscle soreness and that supplementation could be initiated in the ~7 days prior to activity that might cause soreness, such as the preseason or intensified period of strength training [120]. It has also been suggested that n-3 PUFAs may facilitate the transport of red blood cells through the capillary bed, which could lead to enhanced oxygen delivery to skeletal muscle. There is, however, some concern that incorporating omega-3 PUFAs into cell membranes may increase the potential for lipid peroxidation, which is much more evident in athletes who undergo high oxidative stress [121]. Nevertheless, Capó et al. [122] stated that diet supplementation with a functional food rich in docosahexaenoic acid (DHA) reduced the oxidative damage in response to acute exercise in footballers. Recently, omega-3 fatty acid supplementation have been associated with beneficial effects on cardiovascular adaptation to exercise, namely, an improvement of endothelial function, possibly as a consequence of an increase in endogenous production of nitric oxide (NO) [123]. Omega-3 acids may confer a positive effect on exercise, by improving fatty acid delivery to exercising muscles via an increased blood flow. Blood flow improvements are believed to be due to an n-3 mediated suppression of n-6 eicosanoid production. A high n-6/n-3 ratio can lead to elevated levels of pro-inflammatory prostaglandins and thromboxanes. Given that these eicosanoids are pro-aggregatory vasoconstrictors, excessive amounts can lead to reductions in vascular blood flow. Supplementing with n-3 can potentially help to inhibit inflammatory eicosanoid production, as well as interacting with cyclooxygenase in a manner that reduces platelet aggregation, increases dilation of blood vessels, and improves circulatory response [124, 125]. One potential mechanism whereby n-3 supplementation may enhance benefits is via increased lipolysis and β-oxidation. Specifically, n-3 fatty acids are claimed to act as metabolic fuel partitioners, upregulating lipid oxidative enzymes and downregulating lipogenic gene expression [124]. There is evidence that n-3 PUFA supplementation results in increased protein synthesis secondary to n-3-mediated increases in cell membrane fluidity. In addition, n-3 PUFAs may enhance mTOR/p70s6k signaling, a pathway thought be involved in decreasing proteolysis by downregulating the ubiquitin-proteasome pathway [126].
Omega 3 supplementation has a markedly protective effect in suppressing exercise-induced bronchoconstriction, and this is most likely attributed to its anti-inflammatory properties [127]. It may also have an important role in recovery from injury, due to its anti-inflammatory role and its effect on muscle protein turnover. Potentially, n-3 may be helpful during immobilization to ameliorate muscle loss and boost recovery [128–131]. A key point is the question of timing of fish oil supplementation; depending on the timing of the n-3 nonesterified fatty acids (NEFA) consumption (i.e., during disuse or recovery), these compounds could either improve or worsen the muscle atrophy. Different timings targeting different biochemical events (i.e., to decrease oxidative stress/inflammation during immobilization and to increase anabolism during the recovery process) should be also tested [132]. N-3 supplementation, particularly DHA, can also be useful in the recovery process from the neurometabolic insults and structural damage to axons that occur in episodes of mild traumatic brain injury (concussion) [133, 134]. It has also been demonstrated that omega-3 PUFA can affect not only cognitive function but also mood and emotional states (e.g., depression) and may act as a mood stabilizer [135]. Guzman et al. [136] showed that supplementing female elite football players with DHA-rich fish oil for 4 weeks produced perceptual-motor benefits (i.e., improvements in complex reaction time and efficiency), which supports the view that DHA may improve performance in sports where perceptual-motor activity and decision-making are the keys to success. Although, at present, the limited human data do not support the hypothesis that omega-3 PUFA supplementation is effective in enhancing physical exercise performance, there is accumulating data that show improved athlete’s health.
It has been suggested that for most athletes, ingesting approximately 1–2 g/day of EPA and DHA at a ratio of eicosapentaenoic acid (EPA) to DHA of 2:1 would be beneficial in this context. However, it should be noted that an omega-3 PUFA (EPA+DHA) dose of ≤3.0 mg/day has been designated as safe for general consumption by the United States of America Food and Drug Administration (2004) [121]. Within the context of concussions, some current available evidence recommend intake of approximately 400 mg/day DHA [133], while other authors suggest a higher intake (10 mg/kg/day) started right after the concussion [134].
The reported health risks of fish oil supplementation include the (low) risk of contamination with toxins, such as heavy metals, dioxins, and polychlorinated biphenyl (PCBs); there is also the risk of increased bleeding or immunosuppression in case of excessive intake [121].
53.6 Buffers
Despite the fact that sprinting in a football game represents less than 10% of total distance covered, this performance is considered as one of the most critical [137]. The need of performing a sprint during a game varies, and players must be ready to perform, recover, and perform it again at the highest possible level [75].
Brief highly intense muscular effort (short sprints) depends highly on anaerobic glycolysis and leads to large accumulation of lactic acid, which can cause muscle acidification. Because of the body buffering capacity, composed by phosphates, bicarbonate, and carnosine, the H+ concentration remains low even during the most severe exercise, allowing muscle pH to decrease from a resting value of 7.1 to no lower than 6.6–6.4 to exhaustion. Low muscle pH is believed to be the major limiter of performance and the primary cause of fatigue during maximal, all-out exercise lasting more than 20–30s [138]. Evidence shows that fatigue occurs after short-term intense periods in both halves [85].
It may be possible that in circumstances in which there is a great demand on repeated sprint ability, a player performance is limited by muscle acidosis. It appears that repeated sprint ability is related to both intracellular and extracellular buffer capacity. Increased intracellular buffer capacity should help to resist changes in muscle pH, while increases in extracellular buffer capacity may increase H+ efflux out of the muscle cell or otherwise improve physical performance [137].
53.7 β-Alanine
β-Alanine is an amino acid that can be obtained through meat and that can be endogenously synthetized in the liver. By itself, the ergogenic properties of this amino acid are limited; however, β-alanine has been identified as the rate-limiting precursor to carnosine synthesis [139]. Carnosine (β-alanyl-L-histidine) is a cytoplasmatic intracellular dipeptide found in high concentrations in skeletal muscle (10–40-mmol/kg dry weight) [139]. It is estimated that the contribution of carnosine to the total buffering capacity in muscle may be as low as 7% [139], but it is indisputably a functional pH buffer [140]. A second mechanism that is involved in muscle fatigue is the reduction in Ca2+ release from the sarcoplasmic reticulum (SR). A third possible mechanism of performance improvement evoked by carnosine could be related to its antioxidative potential. However, it is clear that further research is recommended to verify the effects of ingestion of β-alanine on oxidative stress [140].
Oral carnosine supplementation is an inefficient method of augmenting muscle carnosine levels in humans, as ingested carnosine is ultimately metabolized before reaching skeletal muscle [139]. The increase of intramuscular availability of β-alanine, through a chronic loading dose, is the more effective way to increase endogenous synthesis of carnosine. Daily doses of β-alanine of 4.8–6.4 g can elevate human muscle carnosine content by 20–30% in 2 weeks [139], 60% in 4 weeks, and 80% in 10 weeks [141, 142]. Employing an incremental strategy ranging from 3.0 to 6.0 g per day may be beneficial [143].
Available literature has been somewhat conflicting regarding the effects of β-alanine on high-intensity performance. When an exercise protocol eliciting an extreme acidosis was used, a significant improvement in performance was observed [142]. On the other hand, when exercise protocols elicit a less extreme muscular acidosis (i.e., a single bout of exercise lasting less than 60s), no effects of β-alanine have been observed [144]. Supplementation improved exercise capacity in tasks lasting 60–240 s, but not in tasks lasting under 60s in which acidosis is not likely the primary limiting factor [145]. Regarding short-duration sprint tasks, carnosine induced no significant improvements in power output in repeated 5-s bouts [146] and a 400-m race [147]. Taken together, research suggests that β-alanine supplementation is capable of improving performance in exercises resulting in an extreme intramuscular acidotic environment, such as multiple bouts of high-intensity short-term exercises, single bouts of high-intensity exercises lasting more than 60s, and single bouts undertaken when fatigue is already present. Exercises with a lower level of acidosis are unlikely to benefit from β-alanine supplementation [140, 148]. β-Alanine supplementation has a discrete ergogenic effect in exercise lasting longer than 4 min [145]. Evidence suggests that there is a small or absent ergogenic effect in endurance performance (lasting 25 min or more) [139], although anaerobic threshold can be shifted after supplementation [148].
A recent study showed that 3 weeks of β-alanine supplementation (4.5 g/d) in college football players did not result in significant improvements in fatigue rates during high-intensity anaerobic exercise, but they suggested a trend for a lower fatigue rate during prolonged (60s) high-intensity exercise. However, as supplementation continued during preseason training camp, subjective measures of fatigue were significantly lower. In addition, training volume tended to be higher in subjects supplementing with β-alanine compared with subjects consuming a placebo [149]. Another study with amateur footballers stated that 12 weeks of β-alanine supplementation improved Yo-Yo intermitent recovery (IR)2 test performance [150]. A study with female footballers showed that 30 days of β-alanine supplementation was able to improve average and peak power when performing consecutive sprints (Wingate test), although lactate did not differ from placebo [151]. Saunders et al. [152] found no effect of β-alanine supplementation (alone or in combination with sodium bicarbonate) on repeated sprints during simulated match play performed in hypoxia.
Although the scientific evidence in untrained populations is substantial, more studies need to be conducted in trained populations and in various sport disciplines in order to fully understand the value of β-alanine supplementation for performance enhancement in elite sports [140].
Daily doses should be divided in doses of 2 g or less in order to minimize side effects. Single large boluses of β-alanine have been shown to induce paresthesia and have not been effective for performance outcomes likely due to strong paresthesia, rapid changes in pH, higher excretion rates, and inability to effectively load the muscle contents. Combining β-alanine consumption with a meal has been shown to be effective for further augmenting muscle carnosine levels [139]. Washout time may vary between non-responders and responders, requiring 6–15 weeks to return to normal [139].
β-Alanine supplementation currently appears to be safe in healthy populations at recommended doses. Paresthesia is the most widely known side effect of β-alanine, being commonly experienced in individuals consuming more than 800 mg of β-alanine in a non-sustained release form. To date, there is no evidence to support that this paresthesia is harmful in any way [139]. It is possible to reach the total daily dose of 6.4 g using 1600-mg doses when using controlled-release capsules. The controlled-release capsules eliminate all symptoms of paresthesia. Four daily doses of 1600 mg may be more practical than eight daily doses of 800 mg and may also ensure greater compliance [148].
53.8 Sodium Bicarbonate
Sodium bicarbonate (NaHCO3) has been investigated as a potential aid to increase the bicarbonate buffer system in blood and interstitium, enhance lactate and H+ translocation from the myoplasm, decrease acid-base balance perturbations, prevent fatigue, and improve performance during high-intensity anaerobic exercise [153].
The exogenous intake of NaHCO3 from 1 to 3 h before exercise results in an important increase in the pH and bicarbonate concentrations in blood at the beginning of an exercise session, as well as during and after it, whereas intramuscular pH and [HCO3 −] remain apparently unchanged [154]. Muscle cell membranes are impervious to HCO3 −, and an increase in extracellular HCO3 − increases the pH gradient between the intracellular and extracellular environment. The effect of this increased pH gradient is to facilitate the efflux of intracellular lactate and H+, thus reducing the fall in intracellular pH [155]. Recently, other mechanism has been proposed, stating that intracellular perturbation may be minimized by the upregulation of Na+/H+ or monocarboxylic transporters or a strong ion difference [156].
The optimal amount should be 0.3-g/kg body weight [157]. Timing sequences with regard to ingestion patterns vary greatly between studies. Attaining peak buffering potential while minimizing the risk of gastrointestinal distress before exercise is essential, especially if athletes are considering loading before an event [158]. For an intake of 0.3-g/kg body weight of NaHCO3, 120 min are needed to reach the peak pH and an average of 100–120 min to reach maximum [HCO3 −] [154]. The substances should be taken in or with fluid, preferably water, and in large quantities (0.5 L or greater).
The ergogenic effect of bicarbonate has been shown in 200-m freestyle swimming [159], 2 × 100-m freestyle swimming (with 10 min of passive rest between bouts) [160], repeated sprints (5 × 6s) or multiple effort bouts observed in recreational team sport [161], and high-intensity intermittent exercise performance (Yo-Yo IR2 test) [162], but not in 200–400-m run [163]. It appears that bicarbonate ingestion can enhance the performance of all-out, maximal anaerobic activities of 1–7-min duration [138].
Subjects should be familiar with possible side effects, which include gastrointestinal distress and, more rarely, gastric rupture, muscle spasms, and cardiac arrhythmias [155]. An alternative protocol can be applied, using chronic NaHCO3 administration [164]. Consumption of 0.5-g/kg body weight during 5 days has ergogenic effect in anaerobic performance (Wingate test) [153]. Chronic ingestion allows subjects to perform more work during high-intensity exercise for at least 2 days after cessation of chronic NaHCO3 supplementation. Moreover, athletes might use NaHCO3 to improve performance without the significant side effects that are usually associated with the ingestion of NaHCO3 in the doses needed. The body may be able to store the [HCO3 −] surplus over a 5-day period and then use it to improve performance during the post-supplementation exercise [153].
53.9 Antioxidants
An antioxidant can be defined as “a compound that in a relatively low concentration, prevents or delays the oxidation of biomolecules” [165]. It is well established that physical exercise results in increased reactive oxygen species (ROS) production in active skeletal muscles. An official football match induces an increased level of oxidative stress and muscle damage throughout 48 h of the recovery period [166]. Dietary antioxidants, like vitamin C, vitamin E, carotenoids, and polyphenols, have received attention predominantly as a nutritional strategy for preventing or minimizing detrimental effects of ROS, which are generated during and after strenuous exercise [167]. Then, antioxidant supplementation has become a common practice among athletes as a mean to reduce oxidative stress, promote recovery, and enhance performance [168].
However, there is still a wide discussion whether antioxidant supplements are helpful or harmful to the athlete [169]. There is no evidence that exercise-induced radical production in skeletal muscle is detrimental to human health. Besides, regular training promotes an increase in the body’s own antioxidant defense system against free radical damage [170]. Until now, requirements of antioxidant micronutrients and antioxidant compounds for athletes competing in different sport events, involving repeated sprinting, have not been determined sufficiently [171].
Actually, the evidence suggests that athletes should use caution when considering supplementation with high doses of antioxidants. A meta-analysis of 68 randomized antioxidant supplement trials (total of 232,606 human participants) concluded that dietary supplementation above the recommended dietary allowance (RDA) with beta-carotene, vitamin A, and vitamin E does not improve health outcomes and may increase mortality [172]. Furthermore, in athletes, antioxidant supplementation can prevent important exercise-induced adaptations in skeletal muscle [173]. Indeed, compelling evidence indicates that exercise-induced ROS production serves as a required signal to promote the expression of numerous skeletal muscle proteins including antioxidant enzymes, mitochondrial proteins, and heat-shock proteins [174]. Some studies in athletes [37–39, 173, 175, 176] indicate that high levels of antioxidant supplementation can blunt the training adaptation to exercise, and one study including football players [177] refers that the antioxidant supplementation group had a lower increase in Vo2max compared with the placebo group.
Further research is required to establish firmly whether antioxidant supplementation is beneficial or harmful to athletes. However, at present, there is limited scientific evidence to recommend antioxidant supplements to athletes. In fact, the consumption of a diet that is nutritionally well balanced can provide a sufficient antioxidant intake, during exercise training, to maintain an appropriate physiological antioxidant status in reference to current recommendations [178].
Some athletes may not consume well-balanced diets, and therefore these individuals could be deficient in antioxidant intake. Prior to the recommendation in using antioxidant supplements, it should be enhanced the importance to nutrition education in athletes. In other situations, moderate and timely limited antioxidant supplementation may be indicated. In cases of high-altitude training periods, it would also be recommended, since radical production is intensified and endogenous defense weakened in hypoxia [179]. Nevertheless, the optimal bioavailability and combined action of multiple phytochemical and antioxidant compounds derived from fruits, vegetables, whole grains, and nuts cannot be replaced by supplementation. In any case, we have to consider the positive and negative effects of free radicals and that the right balance between these and antioxidants is necessary for health and optimal training effectiveness. Antioxidant supplementation may reduce ROS levels, but it is difficult to assume an improvement in athletic performance, only in cases of altitude training [180]. Like other nutritional supplements, it is not a case of “the more, the better.”
53.10 Probiotics
Probiotics are live microorganisms which, when administered in adequate amounts, modify the bacterial population that inhabits our gut and modulate immune function by their interaction with the gut-associated lymphoid tissue, leading to positive effects on the systemic immune system [181]. However, it should be noted that such effects are dose and strain dependent [182]. Probiotics modify the intestinal microbiota such that the numbers of beneficial bacteria increase and usually the numbers of species considered harmful are decreased. Such changes are associated with a range of potential benefits to the health and functioning of the digestive system, as well as modulation of immune function [183]. A recent meta-analysis of the effect of probiotics in respiratory virus infections in the general population showed that 28 out of 33 studies reported beneficial effects [184]. Athletes engaging in prolonged intense exercise may be more susceptible to upper respiratory tract illness (URTI), than individuals participating in moderate exercise or sedentary [185]. In athletes, these symptoms are generally trivial, but no matter whether the cause is infectious or allergic inflammation, they can cause an athlete to interrupt training, underperform, or even miss an important competition [181].
Although there are few published studies of the effectiveness of probiotic use in athletes, there is growing interest in examining their potential to help maintain overall general health, enhance immune function, or reduce URTI incidence and symptom severity/duration. A randomized double-blind intervention study investigated 141 runners taking either a placebo or L. rhamnosus daily during 3 months of training period before a marathon race with follow-up during 2 weeks. Although there were no significant differences in the number of episodes of respiratory or gastrointestinal tract illness, the duration of gastrointestinal symptom episodes in the probiotic group was shorter than in the placebo group during the training period (2.9 vs 4.3 days) and during the 2 weeks after the marathon (1.0 vs 2.3 days) [186]. Another study in elite runners showed that supplementation in L. fermentum decreased the number of days of respiratory illness [187]. A substantial reduction was also observed in URTI incidence among male but not among female athletes after 77 days of L. fermentum supplementation. It remains unclear the differences between the sexes [188]. Probiotic supplementation ingested on a daily basis reduces the number of participants who report any incidence of an infectious symptom in 30 highly trained elite rugby players. The investigators used capsules containing three acid-resistant strains of bacteria (Lactobacillus gasseri, 2.6 billion colony-forming units (CFUs); Bifidobacterium bifidum, 0.2 billion organisms; Bifidobacterium longum, 0.2 billion organisms) [189]. They observed a significant reduction in the number of URTI or gastrointestinal episodes in participants on the probiotic intervention compared to the placebo and significant reduction in the number of days reporting any symptoms during the probiotic treatment compared with a placebo.
Unfortunately there are still no studies of probiotic supplementation in football players, and given the small number of studies that have examined the effects of probiotic supplementation in athletes, it is somewhat premature to issue definitive recommendations. Yet, oral probiotics in athletes, particularly those containing Lactobacillus strains, are a promising area of research. Probiotics may reduce the risk of respiratory and gastrointestinal illness during stressful periods of training and competition. More research is required to clarify issues of strains, dose response, mechanisms, and best practice models for probiotic implementation in the sporting community.
53.11 Vitamin D
Vitamin D is classified as a fat-soluble vitamin but acts functionally as a hormone and has a structure that is similar to steroid hormones. Humans acquire vitamin D from two different sources, endogenous production after sun exposure or via the diet (from food or supplementation). From diet, vitamin D can be found in various food products such as fatty fish, eggs, and dairy products and through various vitamin D analogues produced synthetically in a laboratory. The major source of vitamin D is provided from cutaneous synthesis via exposure to ultraviolet B (UVB) radiation in sunlight [190]. Cutaneous synthesis of vitamin D, however, is dependent on factors including time of exposure, season, latitude, cloud cover, smog, skin pigmentation, sunscreen coverage, and age [191].Vitamin D deficiency is a common but under recognized problem within the global population. The main cause of vitamin D deficiency could arise from the fact that sun exposure be connoted negatively on health. However, moderate sun exposure is the primary means of obtaining vitamin D in humans because it is unlikely to get adequate amounts through diet [192]. Dark-skinned athletes, athletes who live at more poleward latitudes, wear extensive clothing, regularly use sunblock, or consciously avoid the sun, are all at risk for vitamin D deficiency [193]. Although there is no consensus on optimal serum levels of 25(OH)D, vitamin D deficiency is defined by most experts as a total level of less than 20 ng/mL (50 nmol/L). Vitamin D insufficiency is defined as a level of 20–31 ng/mL, and a level of 32 ng/mL (80 nmol/L) or greater is indicative of sufficient levels [194]. Vitamin D inadequacy (serum 25(OH)D <32 ng/mL) was determined in a total of 2313 athletes from different modalities. Fifty six percent (44–67%) of them had vitamin D inadequacy that significantly varied by geographical location [194]. In football players the reality is not very different. A sample of 28 healthy professional football players, white Caucasians, from two First Division teams of Spain provided a blood sample in October and in early February [195]. Two thirds of players had insufficient vitamin D in early February, and almost a third of the group with 25(OH)D above 40 ng/mL in mid-October were below 30 ng/mL in early February. This study suggests that serum 25(OH)D of approximately 48.8 ng/mL is necessary in mid-October to ensure vitamin D sufficiency (30 ng/mL) at the beginning of February. In another study [196], 20 professional male Premier League football players, of varying nationalities, provided a sample in the summer and the winter. Serum 25(OH)D significantly decreased between summer (41.6 ± 8.4 ng/mL) and winter (20.4 ± 7.6 ng/mL) months. All 20 players exhibited a decrease in vitamin D concentration within this time period, where the mean decrement was 21.2 ± 6.0 ng/mL. The two players who were dark skinned exhibited the lowest serum 25(OH)D values in both summer (28 and 27.2 ng/mL, respectively) and winter (8.8 and 15.6 ng/mL, respectively). Also Polish professional football players (n = 24) had lower levels of 25(OH)D after the winter period compared to the summer period (24.96 ± 9.11 ng/ml vs 30.82 ± 9.04 ng/ml) [197]. Finally, in 342 professional footballers based in Qatar, 84% of them had 25(OH)D concentrations less than 30 ng/mL; 12% were severely deficient (<10 ng/mL), and there was a significant difference in 25(OH)D level depending on the country of origin of the player. Total body mass and lean mass were significantly higher in players with 25(OH)D levels greater than 20 ng/mL, when compared with the players with less than 10 ng/mL. However, there was no consistent association found between lower-limb isokinetic peak torque and 25(OH)D concentration [198].
The effects of vitamin D are not limited to bone metabolism. A recent systematic review found a moderate to strong inverse associations between 25(OH)D concentrations and cardiovascular diseases, serum lipid concentrations, inflammation, glucose metabolism disorders, weight gain, infectious diseases, multiple sclerosis, mood disorders, declining cognitive function, impaired physical functioning, and all-cause mortality [199].
The discovery of the vitamin D receptor in human skeletal muscle has led to increased research indicating a direct effect on skeletal muscle activity [200]. It has been long recognized that vitamin D deficiency is associated with muscle weakness, particularly proximal muscle weakness, and that this resolves with correction of deficiency [201]. Evidence from the Russian and German literature at the turn of the twentieth century suggests that UVB exposure makes a positive impact upon athletic performance [193]. However, these studies did not simultaneously measure markers of vitamin D status and were not made using the rigorous scientific standards employed today. Vitamin D affects skeletal muscle by indirect mechanisms, by the change of calcium and phosphate balance, and by direct mechanisms through activation of muscle cells in the vitamin D receptor triggering the transcription of genes involved in differentiation and proliferation of muscle cells [202]. There may be even an influence of vitamin D receptors polymorphisms on musculoskeletal injury. In professional football players, it was found that the ApaI polymorphism may be associated with the severity of musculoskeletal injury [203].
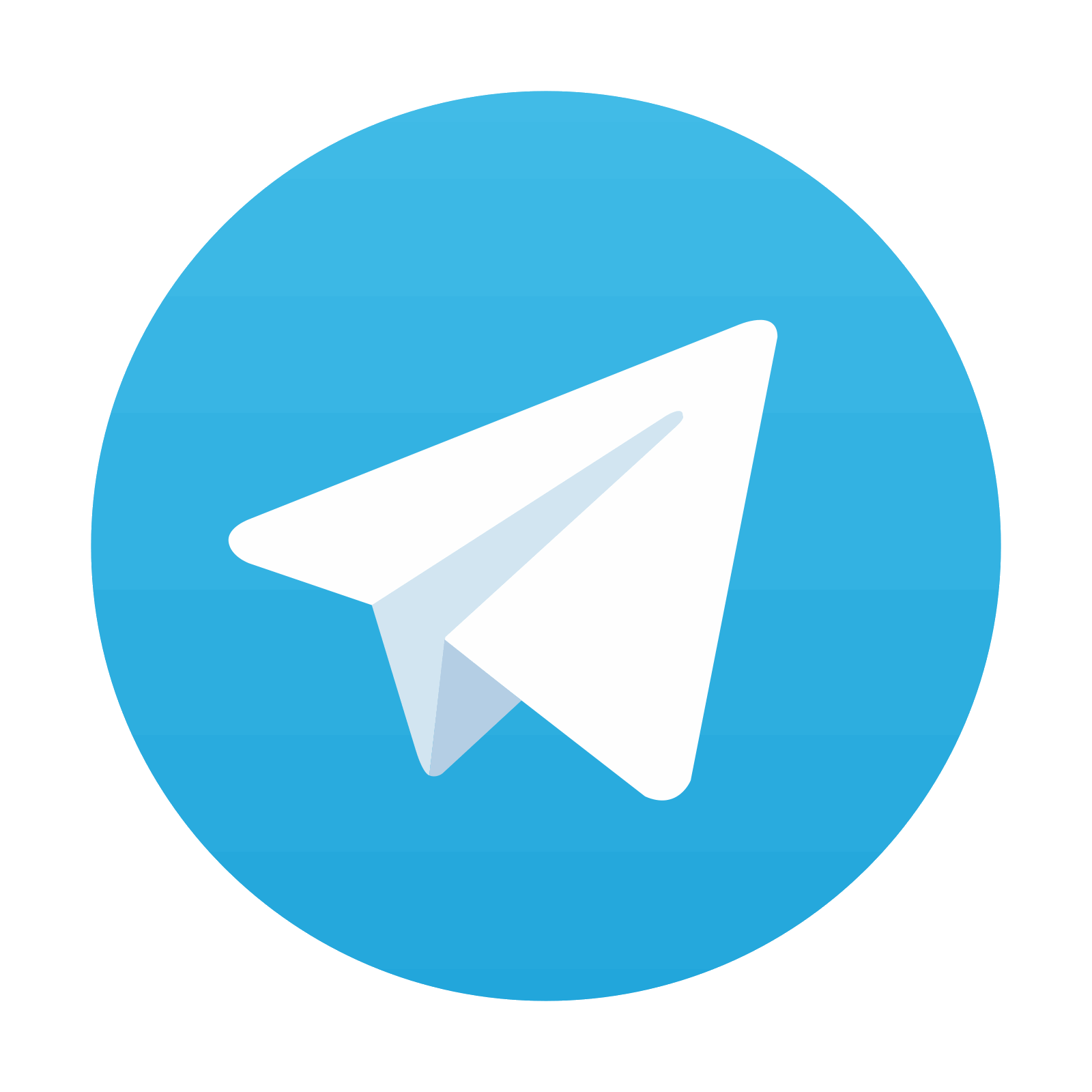
Stay updated, free articles. Join our Telegram channel
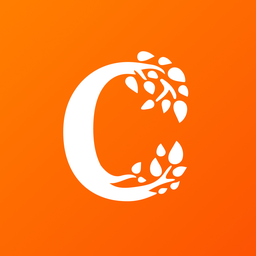
Full access? Get Clinical Tree
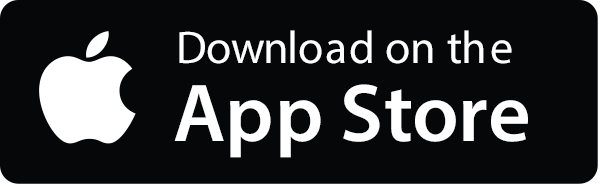
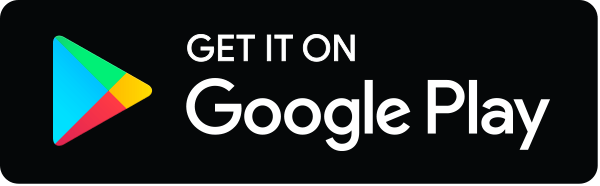