The size and shape of joints markedly affect their biomechanical properties, but the macroscopic 3-dimensional (3-D) mechanism and extent of cartilage and joint maturation during normal growth are largely unknown. This study qualitatively illustrates the development of the bone-cartilage interface in the knee during postnatal growth in humans and C57BL/6 wild-type mice, quantitatively defines the 3-D shape using statistical shape modeling, and assesses growth strain rates in the mouse distal femur. Accurate quantification of the cartilage-bone interface geometry is imperative for furthering the understanding of the macroscopic mechanisms of cartilage maturation and overall joint development.
The load-bearing and compositional properties of articular cartilage vary with both the size and shape of the diarthrodial joint. In skeletally mature animals and humans, the biomechanical and biochemical characteristics of articular cartilage vary by site (eg, patellofemoral groove vs femoral condyles) and joint (eg, knee vs ankle), complementing the local geometry to respond to various loading demands of the body. During postnatal development (the processes of differentiation and growth), joint size increases, and cartilage thickness and chondrocyte density decrease. Concomitantly, articular cartilage load-bearing material properties improve. In animal knees, increases during development in the tensile and compressive moduli of articular cartilage have been associated with increases in collagen content and cross-linking.
The macroscopic mechanisms that dictate cartilage material maturation and joint shaping remain to be determined. At the articular surface, appositional growth occurs with chondrocyte proliferation in the superficial zone and deposition of collagen fibers. Articular cartilage maturation is also affected by changes at the bone-cartilage interface, with chondrocyte proliferation occurring in neonatal rabbit knee joints above the subchondral plate. These macroscopic tissue changes during growth may be described biomechanically by growth deformations and strains. Thinning of cartilage during postnatal development is due to mineralization in the deep zone and advancement of the tidemark, separating the deep and calcified cartilages. In conjunction with these axial growth processes, articular cartilage expands tangentially along with the underlying subchondral bone. Both axial and tangential growth processes may generate internal stresses and affect the material quality of adult articular cartilage. Thus, a detailed understanding of the shape of the bone-cartilage interface during development would provide insight into the biomechanics of cartilage maturation and macroscopic joint size and shape.
The length and shape of long bones during in vivo development is in part contributed through the differential growth and remodeling of articular and growth plate cartilages. Both begin as a homogeneous condensation of chondrocytes that undergo proliferation, hypertrophy, and mineralization in distinct zones to form a highly organized structure at maturity. In the growth plate, rates of chondrocyte proliferation, hypertrophy, and matrix production in different zones have been related to longitudinal bone growth. Differences in these rates at specific locations give rise to differential elongation rates, such as those observed at opposite ends of long bones and between different joints. Additional variations in cartilage growth rates and directions with age and species produce the wide range of joint shapes and relative proportions of anatomic features (eg, lateral and medial condyle proportions in the knee).
Current knowledge of the macroscopic joint shape is based on measurements of cartilage and bone geometries from gross specimens, plain radiographs, magnetic resonance imaging (MRI), and computed tomography (CT). However, the complexities of joint shape are challenging to represent by 2-dimensional (2-D) or best-fit 3-dimensional (3-D) measurements. While the distal femur is characterized by measurements such as anterior-posterior length, medial-lateral width, and intercondylar height, these parameters are confounded by the size of the knee, especially in growing knees, making it difficult to distinguish shape changes during joint maturation. Statistical shape modeling (SSM) is one technique that can concisely quantify complex shapes in a limited number of independent parameters based on a sample population. This method can automatically identify corresponding anatomic regions between samples with high reproducibility, making it ideal for studying shape changes during growth and development. Both 2-D and 3-D SSMs have been used to analyze joint shape and bone density as risk factors for the development of osteoarthritis and osteoporotic fractures, but have yet to be applied to the growth of healthy joints.
The hypothesis of this study was that shape changes of the distal femur at the bone-cartilage interface vary differentially with age and anatomic region during postnatal growth and development. The aims were to (1) illustrate and qualitatively compare the shape changes of the bone-cartilage interface during normal development in humans and C57BL/6 wild-type mice, (2) establish a statistical shape model and determine shape parameters for the growth of the mouse distal femur from postnatal days 12 to 120, and (3) determine growth deformation and strain rates of the bone-cartilage interface. Quantification of the shape plasticity throughout growth and development provides the foundation for investigating in vivo developmental biomechanics of the knee. In addition, quantitative models of the developing knee are useful as design targets for tissue engineering that extends to the joint scale, as well as for the development of new technologies for the clinical diagnosis and treatment of skeletal disorders.
Materials and methods
Sample Preparation and Imaging
With Institutional Review Board approval, clinical CT scans were obtained from 6 patients (range: 3.9–11.9 years; mean: 8.2 years) with tibial torsion abnormalities but morphologically normal distal femora at 0.4 to 0.6 mm in-plane resolution and 0.63 mm slice thickness (GE Lightspeed VCT; GE Healthcare, Piscataway, NJ, USA).
The structure of mouse knee joints was assessed by micro–computed tomography (μCT) and histology. With Institutional Animal Care and Use Committee approval, both hindlimbs of 21 C57BL/6 male mice, n = 3 pairs each at 12, 16, 20, 24, 30, 60, and 120 days postnatal, were harvested, fixed in 10% neutral buffered formalin, and scanned intact by μCT at (9 μm) isotropic voxel resolution (SkyScan 1076; SkyScan, Kontich, Belgium; 70 kVp, 140 μA, 1750 ms exposure). Following μCT, 1 femur at each age point was randomly selected for histologic processing. Proximal and distal femora were decalcified in 10% formic acid and paraffin-embedded. Sagittal sections (5 μm thick) were obtained approximately through the center of the medial femoral condyle of the distal femur. Sections were stained with Safranin-O and digitized at 20× magnification (0.5 μm resolution) with an Aperio ScanScope (Aperio Technologies, Vista, CA, USA).
Gross Morphology
Human and mouse CT images were qualitatively analyzed for morphologic changes at the bone-cartilage interface during developmental growth in 2-D coronal and sagittal planes through the center of the load-bearing region of the condyle and in 3-D reconstructions. Distal femora were assessed for contour of the condyles, posterior condyle prominence, medial femoral condyle (MFC) versus lateral femoral condyle (LFC) size, and trochlear ridge development, as well as location and morphology of the growth plate. Contour of the opposing tibial plateau, as well as joint space width between the distal femoral and proximal tibial secondary ossification centers (SOCs), were also noted.
Histology
Safranin-O sections were compared with matching μCT sections of mouse femora to interpret the bone-cartilage interface of the SOC relative to the overlying articular-epiphyseal cartilage. In addition, histology sections were assessed for chondrocyte hypertrophy and organization.
Image Processing
All CT and μCT scans were imported into Mimics (Materialise, Leuven, Belgium) for surface segmentation and 3-D reconstruction. Left femora were flipped in orientation to match right femora. Bone-cartilage interfaces were identified by thresholding for bone, segmented, and exported as point clouds.
Width and Thickness Measurements
Transepicondylar widths were determined in human and mouse distal femora as a measure of growth. In 3-D reconstructed models, transepicondylar width was measured as the nearest distance from the edge of the lateral epicondyle to the edge of the medial epicondyle. In the mouse, the overall length of the femur was also determined from the most proximal point on the femoral head to the most distal point on the femoral condyles.
Thicknesses of the articular-epiphyseal and growth plate cartilage were calculated from 2-D histology images. Articular-epiphyseal cartilage was defined from the articular surface to the calcified cartilage tidemark, or to the distal edge of large hypertrophic cells for young joints with no tidemark. Growth plate cartilage was defined from the epiphyseal side of reserve zone chondrocytes to the metaphyseal side of terminal hypertrophic zone chondrocytes. Because articular-epiphyseal and growth plate cartilage contours were highly curved in the mouse distal femur, making it difficult to estimate thickness directly, cartilage thickness was calculated as the area of the cartilage divided by the average width of the cartilage.
Statistical Shape Modeling
Both femora of 15 mice, n = 3 pairs each at days 12, 24, 30, 60, and 120, were used as the initial training samples for SSM. Training samples were rigidly registered and isotropically scaled to the largest femur (day 120 sample). Point-to-point correspondences between coordinates of each femur were defined automatically, following previously established methods by first constructing a normalized average atlas shape and then extrapolating points (landmark coordinates), in corresponding locations to each femur. Each distal femur was described by 412 landmarks, located from the femoral condyles to the proximal edge of the trochlea.
The statistical shape model was built from the landmarks of the training samples using principal component analysis. A mean shape was calculated from training sample landmarks, and deviations of each shape from the mean were determined. Singular value decomposition of the covariance matrix was performed to obtain the eigenvectors and corresponding eigenvalues (in descending order). The eigenvectors represent the modes of variation within the training set, analogous to the principal axes of an ellipse. The eigenvalues represent the variance explained by each mode, or the amount of contribution of each mode to overall joint shape variation. Using the modes of variation from the model, the shape parameters, b , of each sample was calculated by X=ˉX+Pb
X = X ¯ + Pb
, whereˉXisthemeanshape
where X ¯ is the mean shape
, P=(p1|p2|…|pt)
P = ( p 1 | p 2 | … | p t )
are the first t modes of variation that explain greater than 90% of the total shape variance, and X is the sample shape. Shape parameters are analogous to principal radii of an ellipse. Parameters were normalized to 1 standard deviation of the mode, calculated as the square root of the corresponding eigenvalue.
Additional samples at days 16 and 20 were analyzed by applying the SSM. Samples were segmented in Mimics, rigidly aligned to the same reference femur from the training set, and nonrigidly aligned to the model atlas shape to extrapolate landmarks. Shape parameters were then determined as described above.
Growth Maps: Deformation Rates, Strain Rates, and Strain Directions
Deformation and principal surface strain rates between ages were calculated from corresponding landmark coordinates of the average shape at each age. Here, landmark coordinates represented non-scaled shapes. Samples were aligned at the centroid of the growth plate to determine deformations during growth. Magnitudes of deformation were calculated as the distance between corresponding landmarks, and deformations in the same direction as the outward surface normal vector were defined as positive. The 2-D components of Green’s strain E ij were calculated from ds 2 − ds 0 2 = 2E ij dX i dX j for i,j = 1:2, where ds 0 2 = dX i dX j is the squared segment length of a pair of landmarks at the first age point, and ds 2 is the squared length at the second age point. Maximum principal strain rates and directions were calculated from the Green strain components.
Deformation and strain rates were mapped in 3-D onto the triangulated surfaces of the average shape at each age point. Surface patches intersecting the transverse plane and sagittal plane through the medial condyle were determined, and the 3-D principal strain directions of those patches were projected onto each plane to illustrate strain directions along the 2-D surface contour.
Statistics
Differences in mode parameters between age points were assessed by a one-way analysis of variance with post hoc Tukey test. All data are expressed as mean ± SD.
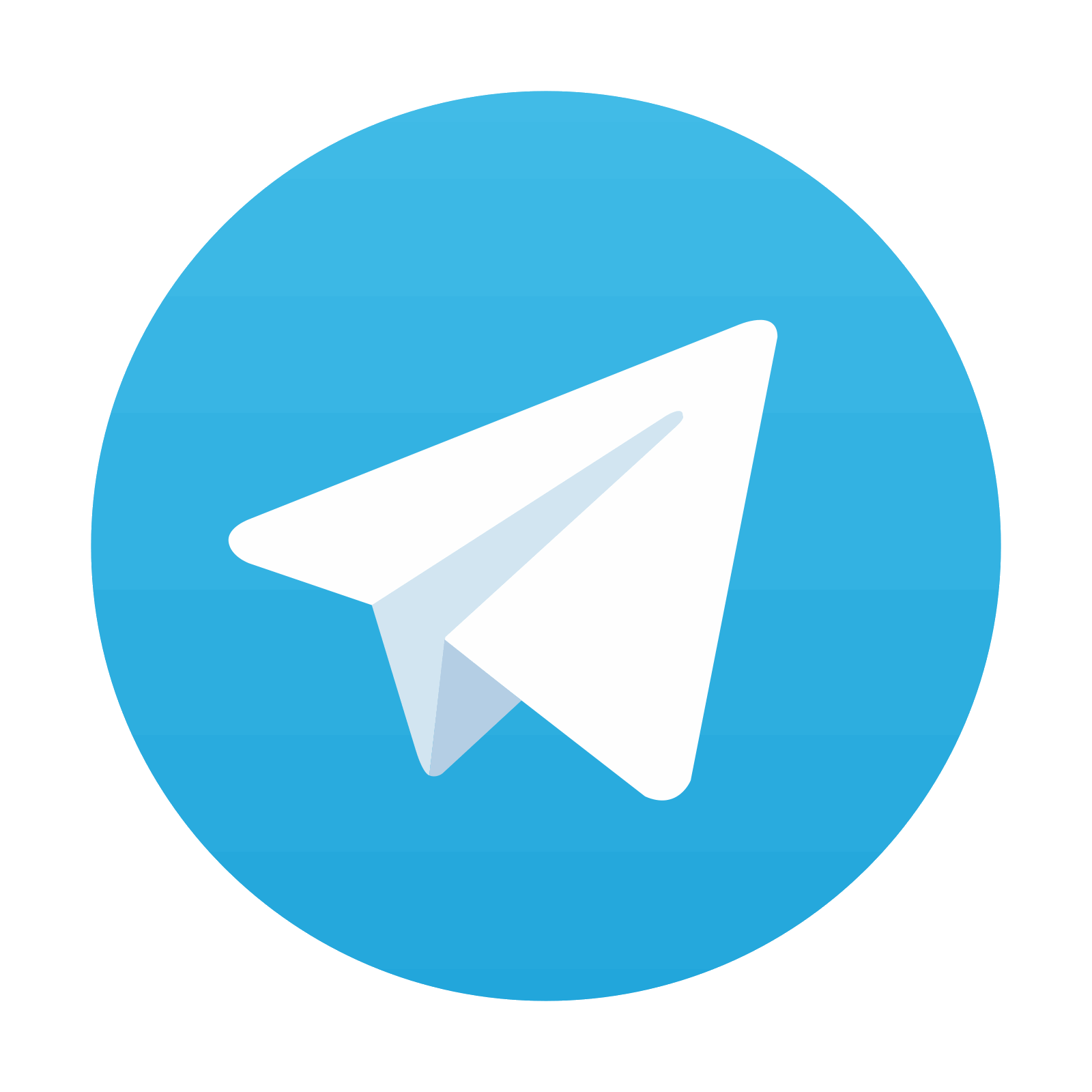
Stay updated, free articles. Join our Telegram channel
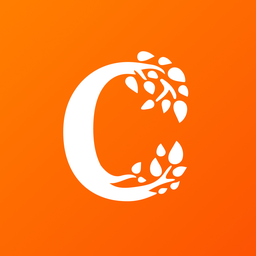
Full access? Get Clinical Tree
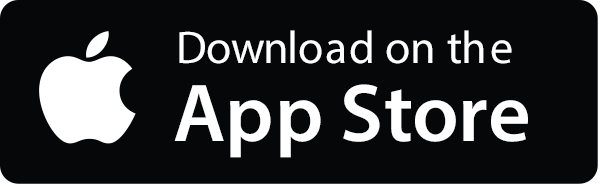
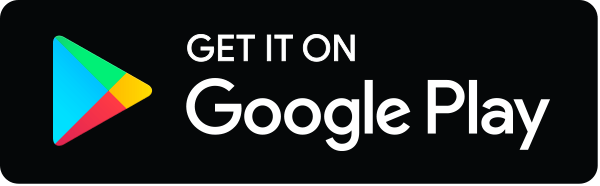