, André Costa6, 7 , Pedro Pessoa8 and C. Niek Van Dijk9
(1)
Clínica do Dragão, Espregueira-Mendes Sports Centre – FIFA Medical Centre of Excellence, Porto, Portugal
(2)
Dom Henrique Research Centre, Porto, Portugal
(3)
Department of Orthopaedics, Minho University, Minho, Portugal
(4)
3B’s Research Group–Biomaterials, Biodegradables and Biomimetics, University of Minho, Headquarters of the European Institute of Excellence on Tissue Engineering and Regenerative Medicine, AvePark, Parque de Ciência e Tecnologia, Zona Industrial da Gandra, 4805-017, Barco, Guimarães, Portugal
(5)
ICVS/3B’s–PT Government Associate Laboratory, Braga/Guimarães, Portugal
(6)
Centro Hospitalar Vila Nova de Gaia/ Espinho E.P.E., Gaia, Portugal
(7)
Clínica do Dragão, Espregueira-Mendes Sports Centre – FIFA Medical Centre of Excellence, Porto, Portugal
(8)
Hospital Ortopédico Sant’iago do Outao, Setúbal, Portugal
(9)
Department of Orthopaedic Surgery, Academic Medical Center Amsterdam, Meibergdreef 9, 1105 AZ Amsterdam, The Netherlands
41.1 Introduction
41.2 Pathophysiology
41.3 Epidemiology
41.4 Risk Factors
41.7.1 Nonoperative Treatment
41.7.2 Surgical Treatment
41.8 Return to Sports
41.9 Future Trends
Keywords
Stress fracturesRisk factorsEpidemiologyManagement41.1 Introduction
A stress fracture is, by definition, a partial or complete solution of continuity in the bone, which results from excessive repeated loads at submaximal intensity, resulting in greater bone reabsorption without equivalent bone ingrowth.
For the correct understanding of the stress fractures, it is necessary to acknowledge the bone biology and its response to stress forces. Bone is a dynamic tissue, which reacts to shear and compression forces, leading to bone transformation according to Wolff’s Law in a constant remodeling and turnover process. It is the disturbance in the equilibrium between bone resorption and regeneration that leads to injury.
A stress fracture was first described by Breithaupt [1] in 1855, as an overuse fracture seen in military recruits. Soldiers reported plantar pain and edema following long marches. In the athletic population, the first clinical description was given by Devas [2] in 1958, based on the results of plain X-rays, correlating the radiological patterns with the pain and impairment.
Stress fractures are among the most common overuse injuries in physically active individuals [3, 4]. These bone injuries can affect almost any bone, although the vast majority of these fractures (up to 95%) will occur in the lower extremity [5, 6]. The most common locations for stress fractures include the tibia, metatarsals, fibula, and navicular bones; less common locations include the femur, pelvis, and sacrum.
It is essential to perform an early and accurate diagnosis, because it will be critical to promptly assess the patient, minimize the period of recovery, optimize athlete performance, and prevent injury relapse. High-risk stress fractures are particularly important to diagnose (i.e., tension side of femoral neck, tarsal navicular, and base of the fifth metatarsal) mainly because of their long recovery period.
Football injuries are commonly divided into traumatic injuries with an acute onset and overuse injuries with a gradual onset [7, 8]. Stress fractures in football players are particularly difficult to diagnose and treat, because of the specificity of the modality and unique psycho-bio-social characteristics of the football player.
41.2 Pathophysiology
Stress fractures can be divided in insufficiency fractures and fatigue fractures. Insufficiency fractures occur when a normal deforming force is applied to the bone with low elastic resistance (i.e., osteoporotic fractures). In contrast, fatigue fractures occur when the bone suffers an exacerbated and repeated or cyclic deforming force, although maintaining its normal elastic resistance.
Intrinsic bone factors that affect the risk of stress fracture include porosity, mineralization, density, trabecular and cortical architecture, and fatigue microdamage, which reduces the elastic modulus.
There are various theoretical models justifying the occurrence of stress fractures. Bennell [9] proposed two different models to explain the appearance of specific fractures. The primary microdamage hypothesis: particular bone sites are maximally stressed, and injury occurs when microdamage is too extensive to be repaired by normal remodeling. Conversely, the primary remodeling hypothesis is based in an accelerated bone remodeling, secondary to genetic factors, bone strain arising from exercise, systemic or reproductive hormones influence, and poor dietary intake. Since osteoclastic resorption always precedes formation in the remodeling process, microdamage may occur at these focal areas of weakness; when the bone is stimulated to submaximal forces, the remodeling process is accelerated, exposing the athlete to temporary periods of local weakness. The fracture will occur when muscle and tendon forces exceed the level of bone resistance. Other theories are based on mechanical factors as rapid load changes or pressure/area changes [11] and negative catabolism due to insufficient dieting [12].
Knowledge of the pathophysiology of bone is essential to understand the multifactorial etiology of overuse fractures that include biomechanical, hormonal, nutritional, and genetic factors. Attention should be paid to the identification of these factors in an attempt to prevent this type of injury in athletes.
41.3 Epidemiology
Stress injuries are common among athletes and military recruits, accounting for approximately 10% of overuse injuries [3].
A number of series of stress fractures among athletes have been described in the literature, but only several reports have described in detail the distribution of stress fractures of the upper and lower extremities and trunk. These studies have generally shown that the tibia is the most common site of stress fractures, followed by the metatarsal and tarsal bones [15–18]. Running activities, especially track and distance running, have been the most common sporting activities that have resulted in stress fractures [16, 19].
These injuries are more frequent in the lower extremity and often related with intrinsic factors such as age, gender, bone alignment, hormonal and nutritional, and bone density and also with extrinsic factors (training, type of shoes, type of modality) [20].
Approximately, 69% of stress fractures occur in runners, although their incidence is not negligible in football players. Aitken et al. [21] preformed an epidemiologic study describing the characteristics of all sports-related fractures; football was the first sports activity causing traumatic bone fractures and accounted for 35.5% of sports-related fractures. In contrast, stress fractures in elite footballers show low prevalence representing only 0.5% of all injuries. Ekstrand study group [8] evaluated prospectively 2379 male football players (54 professional football teams) between 2001 and 2009 and determined an incidence of stress fractures of 0.04/1000 h of football exposure, meaning that an elite team with 25 players in their main squad can expect one stress fracture every three seasons. In the same study, the majority (78%) of stress fractures in elite football players will affect the fifth metatarsal bone. Additionally, it seems there is a higher risk of developing stress injury during preseason training period and that younger players, with juvenile bone structure, are more exposed to stress lesions.
It has been suggested that the sites of occurrence of stress fractures in athletes are activity related and that specific anatomical sites are endemic to certain sports [18, 22–27]. The repeat injury mechanism in football involves rapid changes in direction and rapid acceleration, and de-acceleration movements may be the cause of high-energy bending momentum applied to the fifth metatarsal.
41.4 Risk Factors
Armstrong [28] in 2004 was the first to compare military men and women with stress fracture to uninjured controls matched by gender, age, body mass index (BMI), and preadmission aerobic physical performance and identified sustained negative energy balance as a major risk factor for stress fracture injury, leading to increased muscular fatigue, reduced bone collagen synthesis, and reduced muscular support of the long bones of the lower extremity in recruits. The most common site of stress fracture was the tibia, and female gender experienced a higher relative incidence of stress fractures, probably to concomitant hypoestrogenic state [29, 30].
Other studies [13, 31, 32] showed that the osteoporosis seen in the female athlete triad is a consequence of endothelial dysfunction, and it is related with cardiovascular effects, stress fractures, and musculoskeletal injuries. Female athletes presenting with a stress fracture should always be evaluated for female athlete triad.
Bennell [33, 34] analyzed the ground force reaction during running and concluded that physically active males with smaller bones and less resistance to bending moments are at greater risk of stress fracture than those with larger bones and greater resistance. However, in females this correlation is not linear and may indicate that other risk factors are more important in women.
Intrinsic risk factors include mechanical factors such as bone density, skeletal alignment, and body size and composition; physiological factors such as bone turnover rate, flexibility, and muscular strength and endurance; as well as hormonal and nutritional factors [35]. Extrinsic risk factors include mechanical factors such as practice surface, footwear, external loading, and physical training parameters [36].
Fujitaka [37] conducted a longitudinal study to examine the physical characteristics and environmental factors associated with college football players who sustained a fifth metatarsal stress fracture. In the injury group, toe grip strength was significantly weaker than that in the noninjury group. In addition, the logistic regression analysis suggested that toe grip strength might be associated with fifth metatarsal stress fracture injuries. There was a significantly higher frequency of fifth metatarsal stress fracture injuries affecting the nondominant leg compared with the dominant leg.
Although stress fractures result from repeated loading, the exact contribution of training factors (volume, intensity, surface) has not been clearly established [3–6, 9, 38]. From what we do know, menstrual disturbances, caloric restriction, lower bone density, muscle weakness, and leg length differences are risk factors for stress fracture (Fig. 41.1).


Fig. 41.1
Bone tenderness in the base of the fifth metatarsal
41.5 Clinical Presentation
The clinical onset of a stress fracture is characterized by progressive tenderness, which in an early stage may be indolent and purely neglected, only appearing after escalade in training intensity and frequency. Typically, there is an insidious onset of activity-related pain. Continuing exercise, the pain may well become more severe or occur at an earlier stage of the sports practice [1]. Often, the athlete has a history of an increase or change in the training pattern. Vague symptoms can lead to delayed diagnosis, increase of complications, impairment, and sub-achievement [39, 40]. As the stress reaction progresses to stress fracture, the symptom may turn to persistent pain even during ambulation.
History of the patient’s pain and its relation to exercise is very important to determine the presence of predisposing factors. On physical examination, the most obvious feature is localized bony tenderness. Occasionally, redness, swelling, or periosteal thickening may be present at the site of the stress fracture. Special tests such as the hop test, fulcrum test, and hyperextension test should be performed as indicated by the suspected site. The diagnosis of stress fracture is primarily a clinical one; however, complementary exams will be needed to confirm the injury. Various imaging techniques can be used to confirm the diagnosis and in the majority of stress fractures, there will be no obvious abnormality on plain radiograms.
41.6 Diagnosis and Grading
Diagnosis of stress fractures is a difficult task; it takes clinical presumption, and despite positive clinical exam signs, complementary exams are mandatory. The main quest is to determine the severity and prognosis of the injury [41].
Imaging modalities, including radiography, triple-phase technetium 99 m polyphosphonate bone scanning, magnetic resonance imaging (MRI), computed tomography (CT), thermography, and tuning forks, have been used to diagnose and evaluate stress fractures. Despite the recent advances in diagnostic musculoskeletal ultrasound, its use for stress fracture diagnosis is limited. However, the relative ability of each modality to aid in the distinction of injury severity and prediction of recovery has not been comprehensively addressed. Radiography has been shown to have low sensitivity in the acute phase of bone stress injury, but it is commonly ordered to rule out other abnormalities. Technetium bone scanning historically has been considered the reference standard in the diagnosis of bone stress injury; however, specificity can be low, and positive findings in asymptomatic locations, including positive in athletes considered to be functionally healed, can be clinically misleading. MRI is increasingly recognized to have high sensitivity and specificity for bone stress injury, owing to its ability to depict subtle edema [42]. Reports of diagnosis of stress fracture with CT have also appeared in more recent literature; however, this modality is used less frequently in clinical practice. While bone stress injury grading systems have been reported for radiography, technetium bone scanning, MRI, and CT, their relative relationship to healing time has not been established (Fig. 41.2).


Fig. 41.2
Bone scan presenting stress fracture in the base of the fifth metatarsal bone
There is a negative relationship between clinical severity and time to healing as was the relationship between clinical severity and imaging severity. Clinical and pain assessment is an unreliable marker of healing, and follow-up MRI and symptom resolution may be indicated to guide recommendations for the return to training.
The use of MRI evaluation allows the precise assessment of a repetitive injury to bone by allowing a more accurate diagnosis and the severity at MRI, and time to healing suggests the MRI grading system may have predictive utility (Fig. 41.3).


Fig. 41.3
MRI presenting proximal femur stress fracture in a male football player
Arendt study group [43] showed MRI evaluation had predictive value in estimating the duration of disability, by defining a low grade of stress fracture (i.e., grade 1 and 2) and a high grade of stress fracture (i.e., grade 3 and 4). This grading system might have direct implications in the management of stress fractures, allowing more individualized treatment for the elite athletes [13].
The classification of stress fractures as high risk or low risk is important in the management of such injuries [17, 32, 44, 45]. Regarding prognosis and optimal treatment of a stress fracture cannot be answered without knowing its location, the extent of the structural damage, and the presence or absence of nonunion. High-risk fractures include those likely to progress to complete fracture, delayed union, or nonunion, those that require surgical repair, those that require assisted weight bearing or non-weight bearing, and those occurring on the tension side of the natural biomechanical axis. The sites for high-risk fractures include the fifth metatarsal, the anterior tibia, the tarsal navicular, the femoral neck (tension side), the patella, the medial malleolus, the talar neck, and the first metatarsal sesamoids.
Fractures with a favorable natural history, those that respond well to nonsurgical management, those that allow for unassisted weight bearing, and those that occur on the compression side of the natural biomechanical axis are considered low risk [46]. Sites for low-risk fractures include the femoral shaft, the medial tibia, the fibula, the ribs, the ulnar shaft, the calcaneus, and the first through the fourth metatarsals [47].
Among the most frequently used system is the Fredericson grading system for tibial stress fractures using MRI. The Fredericson grading system [48] defines a grade 1 injury as periosteal edema only, a grade 2 injury as bone marrow edema visible on T2-weighted images only, a grade 3 injury as bone marrow edema visible on both T1-weighted and T2-weighted images, and a grade 4 injury as intracortical signal abnormalities (Table 41.1).
Grade | Radiographic finding | Bone scan finding | MRI finding |
---|---|---|---|
1 | Normal | Poorly defined area | Increased activity on STIR image |
2 | Normal | More intense | Poor definition on STIR and T2-weighted images |
3 | Discrete line | Sharp area of uptake | No focal or fusiform cortical break on T1- and T2-weighted images |
4 | Fracture or periosteal reaction | More intense localized transcortical uptake | Fracture line on T1- and T2-weighted images |
41.7 Current Treatment Options
41.7.1 Nonoperative Treatment
Often the treatment of most stress fractures is relatively straightforward and includes decreasing activity and training intensity and sometimes weight bearing restriction or immobilization. However, patients presenting with high-risk stress fractures, such as displaced femoral neck stress fractures and fifth metatarsal base stress fractures, are more likely to have complications such as nonunion and will need surgical treatment [29, 47, 49, 50].
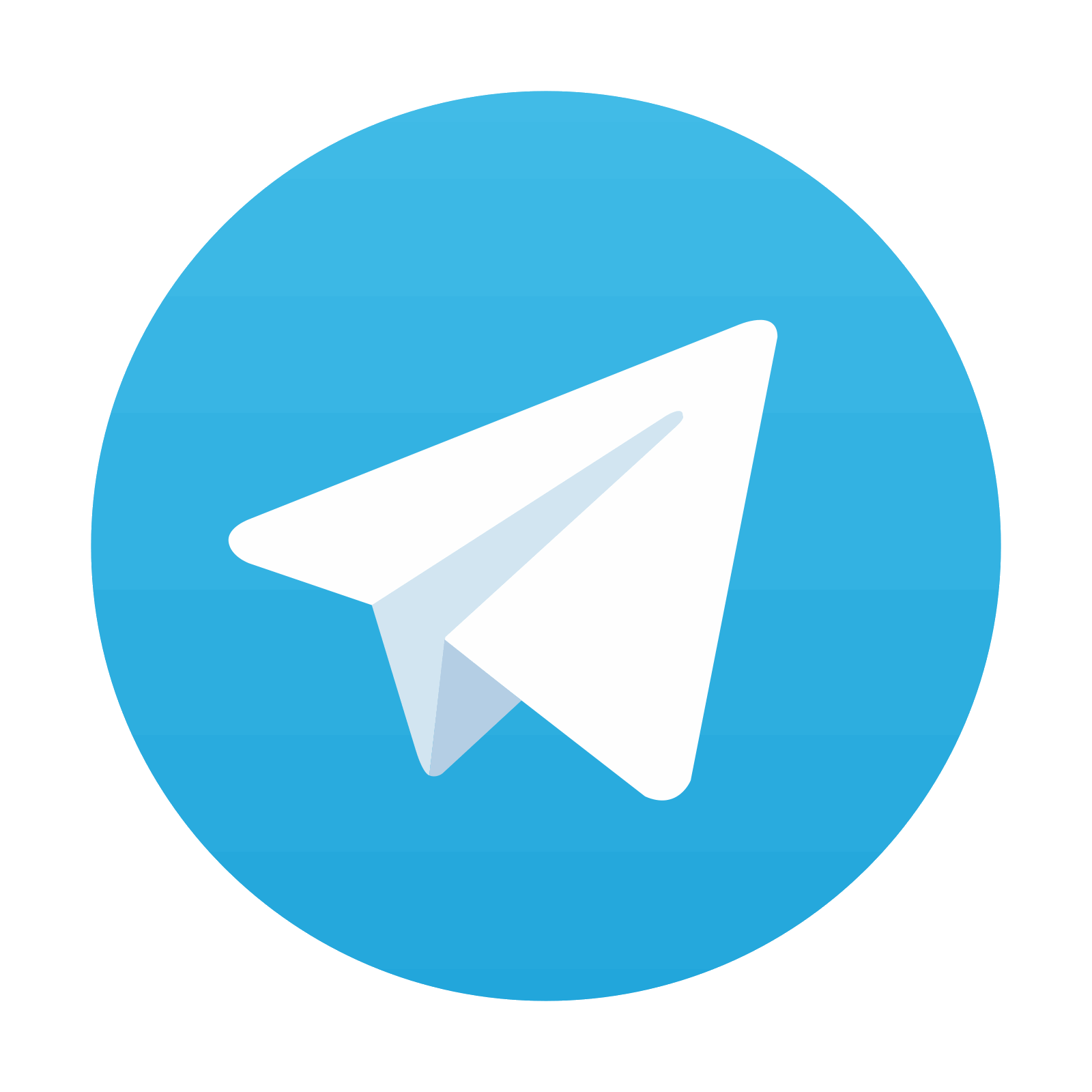
Stay updated, free articles. Join our Telegram channel
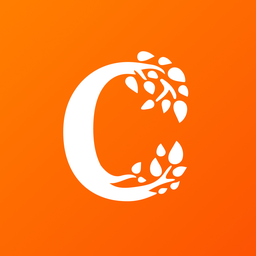
Full access? Get Clinical Tree
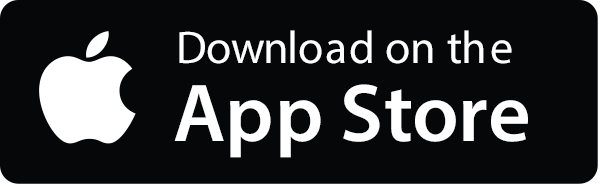
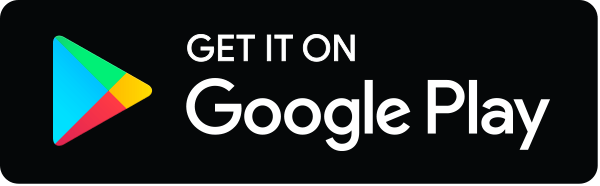