© Springer International Publishing Switzerland 2017
Andrew I. Elkwood, Matthew Kaufman and Lisa F. Schneider (eds.)Rehabilitative Surgery10.1007/978-3-319-41406-5_2727. Spinal Cord and Peripheral Nerve Regeneration Current Research and Future Possibilities
(1)
Rutgers, The State University of New Jersey, New Brunswick, NJ, USA
Keywords
Spinal cordPeripheral nerveNervous systemRegenerationFuture possibilitiesAbbreviations
Akt
Oncogene of transforming retrovirus AKT8 (also Akt1 and PKB)
ATF-2
Activating transcription factor 2
ATF-3
Activating transcription factor 3
BBI
Brain-to-brain interface
BDNF
Brain-derived neurotrophic factor
chABC
Chondroitinase ABC
cAMP
Cyclic adenosine monophosphate
Caspr
Contactin-associated protein
CE
Conformité Européenne
CNS
Central nervous system
CSPG
Chondroitin-6-sulfate proteoglycan
DREADDS
Designer Receptors Exclusively Activated by Designer Drugs
DRG
Dorsal root ganglia
EMG
Electromyography
FDA
Food and Drug Administration
FLAMES
Floating light-activated microelectrical stimulators
GFAP
Glial fibrillary acidic protein
GHBP
Glial hyaluronate-binding protein
HSPG
Heparin sulfate proteoglycan
IGF-1
Insulin-like growth factor-1
IL-1
Interleukin-1
IN-1
IgM antibody that inhibits axonal growth
KSPG
Keratin sulfate proteoglycan (KSPG)
MAG
Myelin-associated glycoprotein
mTOR
Mammalian target of rapamycin
NED
Neural Enhancement Divide
NEP1-40
Peptide 1–40 of Nogo that inhibits NgR
NG2
Neural/glial antigen 2 (includes CSPG4)
NGC
Nerve guidance conduit
NgR
Nogo receptor
NgR1
Nogo receptor 1
NgR3
Nogo receptor 3
NMES
Neuromuscular electrical stimulation
Nogo
Neuronal growth inhibitory molecule in myelin
Nogo-A
The A version of Nogo
Nogo-A/Nogo-B
Nogo-A and Nogo-B genes
NS2.0
Nervous System 2.0
NTR
Neurotrophin receptor (p75 receptor)
OmgP
Oligodendroglial myelin glycoprotein
p75
75 KD protein
PC12
Pheochromocytoma 12
PDGF
Platelet-derived growth factor
PDK1/2
Phosphoinositide-dependent protein kinase 1 and 2
PI3K
Phosphoinositol 3 kinase
PIP2
Phosphoinositol phosphate 2
PIP3
Phosphoinositol phosphate 3
PKA
Phosphokinase A
PKB
Phosphokinase B (also Akt) a serine/threonine protein kinase
PNS
Peripheral nervous system
PTEN
Phosphatase tensin homologue
Rheb1
Ras homologue enriched in brain 1
RhoA
Rho A
RhoK
Rho kinase
RPTPsigma
Receptor protein tyrosine phosphatase sigma
SCI
Spinal cord injury
STAT3
Signal transducer and activator of transcription 3
TSC1/2
Tuberous sclerosis 1 and 2
UCSD
University of California San Diego
Introduction
Our nervous system is remarkable. It not only monitors and controls every part of our bodies but it does so in complex and often elegant ways that exceed our wildest imagination. For example, we know not just the positions but velocities of all our body parts not only relative to ourselves but to the outside world and also our expectations. If you have ever stepped on a moving walkway that is not operating, you may experience a strange feeling and even stumble because you expect the walkway to propel you forward and it does not. The information pouring in through our peripheral nerve system (PNS), vestibular, visual, and olfactory system combine to allow our brain and spinal cord or central nervous system (CNS) to interact smoothly with our environment.
While the brain has been recognized for its momentous processing capabilities, until a few decades ago, we thought the spinal cord to be just a conduit for signals between the brain and the body. We now know that this is wrong and that in addition to substantial processing capabilities, the spinal cord in fact has the ability to learn and interpret information for the brain. So in essence, the spinal cord is a “brain” as well.
The brainstem connects the spinal cord with the brain. It contains the autonomic centers for respiratory and cardiac systems that modulate our breathing and the beating of our hearts, in response to feedback they receive regarding blood pressure, acid, and gas levels (oxygen and carbon dioxide). From second to second, brainstem neurons continually adjust our respiration and cardiac output to maintain our bodies in homeostatic balance, in the face of continually changing physiologic needs and without any conscious effort at all. These brainstem systems connect to the autonomic nervous system that controls skin blood flow and sweating to affect body temperature, mediates vasoconstriction of large blood vessels in the legs that allow you to stand up without fainting, and regulates metabolism.
Another spectacular example of the spinal cord’s independent functionality is seen in “walking circuits” [133]. Over the past two decades, researchers have discovered spinal circuits that, when stimulated, create all the complexities of walking, involving the coordinated control of agonist and antagonist muscle pairs in each limb to create the timed flexion and extension of hip, knee, and ankle joints, and all in reciprocal coordination with the other limb! When we walk, we don’t think of moving our muscles in the sequences of hip flexors, quadriceps, anterior tibialis, gastrocnemius, hamstring, and gluteus maximus on the left and then on the right. Our brain simply tells the spinal cord to walk, and the central pattern generator located in our lumbosacral spinal cord instructs our legs and postural muscles to walk, hop, skip, trot, gallop, or run. Initially described by Miller and Scott in a feline model, this mechanism is now being used to help humans learn to walk again and rebuild their muscles after spinal cord injury (SCI) [227, 228, 338]. Our automated walking patterns may in fact be “simply” ingrained in highly tuned spinal circuits, so that our brains really only need to change the gain on these (inhibit or stimulate them) to influence pace and provide more detailed control for more specific tasks such as dealing with perturbations (e.g., tripping), climbing stairs or rocks, or walking on an uneven terrain or across a beach. The same is true when we micturate, defecate, and ejaculate. In fact, our brain does not control these activities explicitly, as we all know, when we need to go to the bathroom and our brain has a hard time inhibiting the urgency.
In 1906, Santiago Ramón y Cajal opined that “once the development has ended, the founts of growth and regeneration of axons and dendrites dried up irrevocably,” as translated by Raoul May [140, 262]. This opinion by Cajal, the revered “father of neuroscience,” influenced many generations of investigators. For much of the last and current century, scientists assumed that the central nervous system not only does not but cannot regenerate. In contrast, scientists assume that peripheral nerves can and do regenerate. For the last three decades, regeneration research focused on why the central nervous system cannot regenerate while the peripheral nervous system can.
We hope the following will show that these ideas are too simplistic and misrepresent the ability of both the CNS and the PNS to recover from injury. In the case of CNS injuries, clinicians are deeply pessimistic about the possibility of recovery and often express this pessimism to their patients. In the case of PNS injuries, clinicians are sometimes overoptimistic in their prediction of recovery and do not express the limitations of recovery, particularly after delayed or long gap repairs. Finally, the future of repairing both the spinal cord and peripheral nerves is bright. Scientists are considering not only restoring function to normal but propose even improving function beyond normal. Combinations of advanced technology may enable patients to perform better in some respects than before injury.
Spinal Cord Regeneration
Three theories dominate neuroscience today concerning why the central nervous system cannot regenerate while the peripheral nervous system can. The first is axon growth inhibitory effects of the myelin-associated protein Nogo [283, 296]. The second is glial scar, i.e., that astrocytes form a barrier to axonal growth [268, 273]. The third emphasizes extracellular matrix proteins produced by glial cells, particularly chondroitin proteoglycans [35, 80, 86, 109, 214]. All three theories are based on the assumption that the spinal cord cannot regenerate and that peripheral nerve can regenerate without difficulty. This assumption has unfortunately become a self-fulfilling prophecy. Despite much evidence indicating that the spinal cord can regenerate, investigators chose spinal cord models that cannot regenerate to substantiate their assumption and ignored evidence that the spinal cord can regenerate. Recent research indicates that axons can regrow in the spinal cord despite glial scars, Nogo, and chondroitin proteoglycans and that peripheral nerves do not regenerate as well as usually assumed [357].
Early Studies of Spinal Cord Regeneration
In the nineteenth century, several European investigators studied injured animal spinal cords. In 1874, Eichhorst and Naunyn crushed the spinal cords of rabbits and dogs and described many nerve fibers that “infiltrated with neuroglial tissue after three to five weeks” [91]. They saw modest improvement of function. In 1876, Schiefferdecker severely criticized the study by Eichhorst and Naunyn [281]. He found no evidence of regeneration or functional return in dogs up to 300 days after spinal cord transection, claiming that they needed to transect the spinal cord and that any apparently recovered function was due to residual axons or reflexes.
In 1906, Ramon y Cajal largely resolved the issue by transecting the spinal cord of young cats and dogs and found that large numbers of severed intraspinal fibers sprouted new processes with growth cones similar to those observed in transected peripheral nerves [262]. After several weeks, however, the growth slowed and became abortive. He and his colleagues proposed that central nerve fibers commenced regeneration like the peripheral nerve but, for reasons that were not clear, did not continue across the transection site and did not make functional connections in the opposite stump [263]. In contrast, he described robust regeneration of peripheral nerves. The matter of regeneration rested there for several decades.
In the 1920s and 1930s, several investigators reported regeneration in the spinal cord of tadpoles, newts, and young rainbow trout [138, 139, 210]. As Sugar and Gerard pointed out in 1940, regeneration in the central nervous system of nonmammalian vertebrates was generally accepted by the 1920s [204, 311]. Many investigators studied regeneration in lower vertebrates in hopes of finding differences between mammalian and nonmammalian spinal cords that would explain the inability of the former to regenerate [30, 61]. After World War II, when the advent of antibiotics and better bladder care allowed long-term survival of many spinal-injured patients, many investigators began to study treatments to regenerate mammalian spinal cords.
By 1980, the field had divided into those who believed in regeneration and those who did not [344]. The latter dominated. In 1980, Guth et al. reported that enzyme therapy does not regenerate transected rat spinal cords [119]. In that study, they found that if they left even a small strand of spinal cord in the ventral side of the spinal cord, some of the rats recovered walking. So, they proposed a “string” test, recommending that investigators slip a string under the spinal cord and pull it up to confirm transection. The string test ensures a gap between cut ends of the spinal cord and requires transection of the anterior spinal artery, which caused ischemic necrosis of the stumps. A gap and ischemia virtually guarantees no regeneration. Some investigators even sought to find ways to eliminate dorsal root sensory fiber growth so that they could study “true central axonal growth” [315]. Those who did not believe used models to ensure growth does not occur.
In the mid-1980s, several scientists began putting bridges into the spinal cord in the belief that axons need something to grow on. In 1984, de la Torre put a collagen matrix bridge into the spinal cord and found that central neurites and catecholaminergic axons extended across the bridge into the distal stump, albeit without functional recovery [76]. In 1985, Reier et al. began to use a variety of neural tissues to bridge the SCI site, finding that fibers would grow into fetal tissues used in this way, and may even extend beyond, but without functional recovery [266].
Other investigators, such as David and Aguayo and Carlstedt et al., were fascinated by the growth of fibers out of and into the spinal cord [49, 50, 74]. When peripheral nerves were inserted into the spinal cord, fibers grew out of the spinal cord to travel long distances in the peripheral nerve. Based on these results, David and Aguayo hypothesized that spinal fibers are able to grow long distances in peripheral nerve, but that something in the central nervous system environment prevented their growth in the spinal cord. The hypothesis initiated a worldwide search for axonal growth inhibitors.
Go or Nogo?
In 1990, Savio and Schwab reported that lesioned corticospinal tract axons regenerate in demyelinated rat spinal cords [277]. Schnell and Schwab found that corticospinal axons will regenerate in rats treated with an implanted hybridoma secreting an IgM antibody (IN-1) against myelin [283]. This finding initiated intensive efforts to identify the responsible myelin protein [11, 18, 102, 106, 136, 137, 280, 288, 289, 324]. Schwegler et al. confirmed that demyelination allows regeneration in the spinal cord [296]. Others showed that the IN-1 stimulates regeneration and plasticity [36, 39, 42, 102, 117, 170, 182, 261, 272, 287, 290–293, 314, 322, 323, 327, 334, 336, 362]. Immunization of animals against myelin promoted axon growth [300].
In 1998, Schwab et al. isolated a myelin protein that inhibited axonal growth [57, 307]. Called Nogo, the molecule belongs to the reticulon family of proteins [12, 57, 115, 143, 241]. Antibodies against Nogo enhanced regeneration and reorganization of the brain and spinal cord [196, 211, 233, 337]. Discovery and characterization of the Nogo receptor soon followed [17, 37, 77, 100, 146–148, 218, 252]. The Nogo receptor (NgR) provided insights into how Nogo may work. Several myelin proteins bind the Nogo receptor, which acts through the intracellular messenger rho A (RhoA) and rho kinase (RhoK), to regulate microtubule formation essential for axon growth [13, 17]. Increasing intracellular messenger cyclic adenosine monophosphate (cAMP) inactivates RhoA and blocks effects of Nogo and other growth inhibitors. Fournier et al. found that RhoK inhibition enhances axon regeneration in chick dorsal root ganglia (DRGs) [101]. NgR activation not only inhibits neurite growth but cell spreading as well [244]. NgR blockade promotes recovery after SCI [193, 194].
Nogo turned out to be more complicated than anybody suspected. First, there are three Nogo proteins: A, B, and C. Genetic knockout of Nogo-A promotes sprouting [302]. Only Nogo-A binds NgR, but Nogo-C delays regeneration, and adult mice lacking both Nogo-A and Nogo-B sprout corticospinal tracts and recover more function than mice that lack Nogo-A alone [171]. Nogo-A interacts with Nogo-B and -C, as the well as paranodal axonal protein Caspr [84, 240]. Second, neurons express Nogo-A not only on their surfaces but in nuclear chromatin, cytoplasm, and postsynaptic zones [154, 199]. Nogo-A expression increases with neuronal activity [160, 222]. Schwann cells also express Nogo-A [255]. Third, Nogo affects more than just neuronal growth. Nogo-A modulates experimental autoimmune encephalomyelitis, regulates hippocampal neuronal architecture, inhibits angiogenesis, and redistributes protein disulfide isomerase [98, 328, 353, 363]. Nogo-B suppresses tumors and induces apoptosis in cancer cells, regulates hepatic fibrosis, is necessary for macrophage infiltration, and interferes with mitochondria-endoplasmic reticulum interactions [258, 312, 359, 365].
NgR turned out to be complicated as well. NgR exists in a complex with p75 receptor (NTR), the low-affinity neurotrophin receptor [331]. The NgR/NTR receptor complex binds at least three known axon growth inhibitory proteins: Nogo-A, OMgP, and MAG [45, 186]. However, multiple receptors mediate the effects of these growth-inhibiting proteins. For example, MAG inhibition of neurite outgrowth may not require NgR [348]. For DRG neurons, NgR mediates most MAG inhibition because it is reversed by phosphatidyl-specific phospholipase C, which cleaves glycosylphosphatidylinositol anchors, or NEP1-40, a peptide inhibitor of NgR. However, gangliosides mediate MAG inhibition in cerebellar granular neurons because sialidase or ganglioside synthesis inhibitors block MAG effects [221, 343]. Finally, NgR is a family of receptors and NgR1 is the one that binds Nogo.
Genetic results have been mixed. Mice lacking Nogo-A have increased sprouting, enhanced motor coordination and balance, and more spontaneous locomotor activity after spinal cord transection, but the effects were strain dependent [82, 302, 341]. Genetic knockouts result in less regeneration than blocking Nogo or Nogo receptor [317]. Genetic knockout of NgR1 impairs cognitive outcomes, but inhibition of NgR1 enhances cognitive function after brain injury [126, 319]. NgR1 knockout and NgR1 decoy treatment (administering a soluble NgR1 receptor that binds Nogo so that it cannot bind NgR on cells) improve recovery in SCI [320, 332]. Kim et al. reported Nogo-A/Nogo-B knockout mice sprouted corticospinal tracts rostral to spinal cord transection sites and recovered locomotor function [172].
Glial Scar
For many years, scientists knew that “glial scars” formed in brain and spinal cord but did not think that they prevented regeneration [23, 256, 257]. For example, in 1979, Reier described regenerative optic nerve axons that penetrated “extremely dense” glial scars in Xenopus tadpoles and concluded that mature hypertrophic astrocytes do not represent a major obstacle to axonal outgrowth [264]. Matthews et al. described axons crossing the glial limitans into glial scar in transected rat spinal cords [215]. In 1981, Guth et al. found “extensive regeneration” of intrinsic spinal cord and dorsal root fibers in transected spinal cords of hibernating squirrels, concluding that mammalian spinal cord neurons have considerable regenerative potential and that “mechanical impediments such as collagenous and glial scarring, cyst formation, and cavitation” cannot be the sole explanation of why regeneration in mammalian CNS is abortive [120]. In 1983, Shreyer and Jones reported that corticospinal axons, in many cases, grow past both thermal lesions and sharp sections of the spinal cord despite glial scars [286].
By the mid-1980s, much evidence suggested that glial scars block regeneration. In 1986, Borgens et al. found that dorsal column axons seldom grew into a glial scar of a transected spinal cord [31]. Smith et al. showed that a critical period exists after injury during which reactive glia will not support axon growth [303]. In 1984, Kastner assessed axonal growth through astrocytes in fish, finding that astrocytes without orthogonal arrays of particles allow axons to grow through, but astrocytes with such arrays will not [346]. In 1988, Houle et al. described foci of dense confluent neuropil crossing the injury site of chronically injured spinal cord, wherever openings of glial scar occur, suggesting that glial scar does obstruct axonal growth [142, 268]. However, Dahl et al. described axons growing through old multiple sclerosis plaques, concluding that reactive astrocytes do not constitute non-permissible substrate for axon growth [67]. Mansour et al. suggested that astrocytes expressing glial hyaluronate-binding protein (GHBP) permit axon growth [212].
In 1990, Rudge and Silver studied neurite outgrowth into astroglial scars in vitro [273]. They found that glial scars stimulated minimal neurite outgrowth compared to other cells or tissues and proposed the glial scar theory. Bovolenta et al. found that growing neurites avoid anisomorphic reactive gliosis but neonatal glia stimulate neurite outgrowth and concluded that astrocytes both stimulate and inhibit axonal growth [33, 34].
Many subsequent investigators studied glial scars to understand how they form. For example, Guilian et al. examined the effects of microglia-derived cytokines on brain gliosis, finding that the proinflammatory cytokine interleukin-1 (IL-1) is a potent microglial mitogen and regulates microglial components of the glial scar and that IL-1 supported neuronal growth through the glial scar [112]. Frisen et al. observed that pheochromocytoma-12 (PC12) cells do not adhere to spinal cord sections taken rostral to a dorsal funiculus section even though no myelin is present in these areas, suggesting that glial scars lack critical cellular adhesion molecules [103]. In 1997, Davies et al. found that transplanted dorsal root ganglion neurons grow long distances in myelinated white matter tracts but stop at cuts of the spinal cord where a glial scar is present, suggesting that the main obstacle to regeneration is the glial scar and not myelin-based inhibition of axon growth [75].
A popular approach to glial scars is to bridge them with fetal tissues and peripheral nerves, even though such transplants stimulate gliosis and even glial scars [2, 9, 64, 73, 142, 164, 217, 265, 267, 279]. Some investigators reported that axons seldom entered into scar without accompanying astrocytes, suggesting that astrocytes guide axonal growth [24, 95, 121, 123]. Gliosis is essential for repair of the blood-brain barrier [269]. In 2005, Hermanns et al. reported that iron chelation and a collagen synthesis inhibitor reduced scar formation in spinal cord [131]. Klapka et al. subsequently reported that iron chelation and cAMP delayed fibrous scarring, promoted corticospinal tract growth, and improved functional recovery in SCI [173].
Proteoglycans
In 1991, McKeon et al. found that neurite outgrowth in glial scars correlated inversely with expression of inhibitory molecules on reactive astrocytes, particularly chondroitin sulfate proteoglycans (CSPG) independently of glial fibrillary acidic protein (GFAP) production [220, 330]. In 1994, Levine et al. described increased expression of the neural/glial antigen 2 (NG2) family of CSPG after brain injury [191]. Proliferating NG2-positive cells clustered around the lesion, peaked at 7 days, and then declined. Concurrently, microglia activated and increased in number, monocytes invaded the injury site, and an astrocyte scar formed. He proposed that these NG2-positive cells prevent axonal growth in the central nervous stem. In the same year, Jones et al. showed that macrophages and oligodendrocyte progenitor cells express NG2 [157].
In 2002, Bradbury et al. reported that a bacterial enzyme called chondroitinase ABC (ChABC), that broke down CSPG in injured spinal cords, promotes functional recovery after SCI in rats [35]. Many investigators subsequently confirmed beneficial effects of treatments that break down or reduce CSPG production [32, 79, 93, 113, 116, 125, 152, 161, 195, 360, 361]. In 2006, Barritt et al. reported that ChABC promotes sprouting in intact and injured spinal cords [16]. In 2007, Cafferty et al. found functional regeneration through astrocytic scar genetically modified to digest CSPG [44]. In 2009, Nakamae et al. found ChABC promotes corticospinal tract growth in organotypic cocultures [234]. Lee et al. showed that thermostabilized ChABC enhanced axonal sprouting and improved recovery [189]. In 2011, Carter et al. showed that delayed ChABC treatment reverses atrophy of rubrospinal neurons [55]. Wang et al. found that ChABC therapy and rehabilitation promotes forelimb recovery of rats with chronic SCI [333]. Zhao et al. transfected animals with ChABC expressing lentiviral vectors and found better recovery [367]. In 2012, Starkey et al. showed that ChABC promoted compensatory sprouting of remaining corticospinal axons and improved forelimb function after unilateral pyramidotomy [308]. In 2015, Mondello et al. found that 4 weeks of ChABC treatment promoted earlier recovery in cats after SCI [232].
Some beneficial effects of ChABC may be due to mechanisms other than regeneration. Garcia-Alias et al. found that ChABC injected into C5 spinal cord lesions improved forelimb functional recovery by increasing plasticity [108]. Orlando et al., however, showed that full digestion of motor cortex CSPG by ChABC aggravated motor deficits, suggesting that too much plasticity is not beneficial [246]. Milbreta et al. found that ChABC altered astrocytic and vascular structure in injured spinal cords [226]. ChABC also promotes remyelination [185].
Krautstrunk et al. found increased keratin sulfate proteoglycan (KSPG) in injured spinal cords and proposed that KSPG contributes to poor growth of corticospinal tract axons in injured spinal cords [178]. Ito et al. found that mice deficient in N-acetylglucosamine 6-O-sulfotransferase-1 lack 5D4 reactive keratin sulfate in the CNS and that contusions of these mice at the T10 spinal cord level resulted in better locomotor recovery than in control mice [151]. Ishikawa et al. recently showed that combination of keratin sulfate and chondroitin sulfate digestion followed by rehabilitation is better than either alone or without rehabilitation [150].
In 2005, Sapieha et al. reported that the receptor protein tyrosine phosphatase sigma (RPTPsigma) inhibits retinal ganglionic axon growth [275]. In 2010, Duan and Giger proposed that KSPG inhibits axon growth by binding RPTPsigma [86]. Fry et al. soon showed that mice deficient in RPTPsigma regenerate their corticospinal tracts [104]. Coles et al. found that RPTPsigma acts bimodally in sensory neurons, with KSPG inhibiting growth while heparin sulfate proteoglycan (HSPG) promotes growth [63]. In the meantime, Dickendesher et al. reported that NgR1 and NgR3 are receptors of KSPG [80]. In 2014, Zhou et al. showed that reducing RPTPsigma with lentiviral shRNA promotes neurite outgrowth and improves recovery in rat spinal cord contusion [369]. Xu et al. showed that RPTPsigma inhibition enhanced corticospinal and serotonergic axonal growth [352]. Dyck et al. showed that KSPG binding of RPTPsigma inhibits neural stem cell growth, attachment, survival, proliferation, and differentiation [88]. Lang et al. found that blocking RPTPsigma improves connections and functional recovery [183].
One, Some, or All of the Above?
Several key negative studies encouraged many investigators to believe that none of the growth inhibitors are solely or even primarily responsible for regeneration failure in SCI. In 2003, Jones et al. studied axon growth through CSPG, NG2, brevican, neurocan, versican, and phosphacan deposits at interfaces of NGF-secreting fibroblast transplants in injured spinal cords [158]. Many axons penetrated this inhibitory milieu to the fibroblast grafts, including dorsal column sensory fibers, rubrospinal, and nociceptive axons. The axons grew on NG2 especially when the cell adhesion molecules L1 and laminin were present. They concluded that local permissive molecules can balance or exceed inhibitory signals from KSPG. In 2007, Hossain-Ibrahim et al. studied SCI in mice that do not express NG2 [141]. In theory, these animals should show better regeneration, but they did not differ from wild-type mice in their regenerative response or recovery after SCI. They concluded that NG2 is not a major inhibitor of axonal regeneration after SCI. In the same year, Lu et al. found that axons readily penetrated glial scars and deposits of KSPG and NG2 in chronically injured spinal cord transplanted with bone marrow stromal cells, especially in the presence of NT-3 [205]. In 2010, Lee et al. systematically deleted Nogo, MAG, or OMgP [190]. Deleting one of the three inhibitors enhanced corticospinal or serotonergic axonal sprouting without behavioral improvement. However, deleting all three inhibitors was not synergistic. They concluded that Nogo, MAG, and OMgP modulate axon sprouting but do not play a central role in CNS axon regeneration failure.
Many investigators consequently believe that multiple growth inhibitors must be blocked and that growth factors should be provided for regeneration to occur. For example, Schwab et al. gave NT-3 alongside the Nogo antibody IN-1 [36, 114, 284, 285, 327]. Others used IN-1 with acidic FGF-fibrin and with fetal transplants [117, 287]. Liu et al. used recombinant adenovirus to introduce multiple therapeutic genes [197]. In 2013, Hunanyan et al. found that combination of ChABC and AAV-NT3 was synergistic in promoting neural plasticity of descending spinal pathways after SCI [145]. Karimi-Abdolrezaee et al. showed that ChABC and growth factors enhanced endogenous neural precursor differentiation and functional recovery after SCI [168, 169]. In 2014, Alluin et al. found that ChABC, neurotrophins, and locomotor training enhanced sprouting of corticospinal and serotonergic fibers, attenuated astrogliosis and inflammation, and improved walking recovery in rats [5]. The authors noted that growth factors increase KSPG production by astrocytes [304].
Interestingly, ChABC is synergistic with many therapies but not with Nogo receptor blockade [368]. For example, Nakamae et al. found that ChABC and NEP1-40 (a Nogo receptor blocker) each improved corticospinal tract growth, but the combination did not have synergistic effects [235]. ChABC is synergistic with many cellular transplant therapies, including immature astrocytes, Schwann cells, and olfactory ensheathing glia [43, 97, 99]. Kanno et al. found that Schwann cells genetically modified to secrete neurotrophin and chondroitinase promote axonal regeneration and locomotion in rats [166].
Bridging: Many investigators use peripheral nerve, scaffolding, or other bridges, to bypass the lesion site, although it is questionable whether bridging alone can completely avoid myelin-based inhibitors, glial scar, and inhibitory extracellular matrices at spinal cord interface to the bridges [181]. For example, Guest et al. used channels containing Schwann cells to recruit axons and direct the axonal growth to spinal cord below the injury site [118]. However, while the axons grew into the channel, they were reluctant to grow out of the channel into the spinal cord. Liu et al. used collagen tubes to bridge spinal cord directly to nerve roots, showing that many spinal cord neurons sent axons into these tubular implants to reinnervate muscle [198]. Kaneko et al. used a nanofibrous hydrogel combined with a honeycomb collagen sponge to repair completely transected spinal cords and improve locomotor recovery [165]. Most of these therapies, however, achieved only modest regeneration of long tracts with limited and often nonfunctional locomotor recovery.
None of the Above
Some investigators claim to have achieved regeneration in the spinal cord even though they did not address any of the known inhibitors and obstacles to regeneration. In 2005, Tsai et al. reported corticospinal tract regeneration into lumbar gray matter, correlating with locomotor recovery, after complete transection and repair with peripheral nerve grafts, fibroblast growth factor, and fibrin glue, together with spinal fusion [321]. Novikova et al. reported regeneration after neurotransplants combined with brain-derived neurotrophic factors (BDNF) [242]. Oudega et al. combined insulin-like growth factor-1 (IGF-1) and platelet-derived growth factor (PDGF) alongside Schwann cell grafts [247].
The best regenerative therapies appear to be ones that do not involve blockade of any of the growth inhibitors. In 2012 Lu et al. from the Tuszynski laboratory at the University of California, San Diego (UCSD), published two papers reporting massive regeneration in the spinal cords of rats [206, 207]. The first study examined rats treated with cAMP injections into the brainstem, bone marrow stromal cell grafts in the lesion site, and virally induced BDNF expression beyond the injury site [208]. This combinatorial therapy resulted in massive motor axon regeneration beyond both C5 hemisection and T3 complete transection sites, formation of many synapses with neurons below the injury site, but paradoxically worse locomotor function. The second study assessed long-distance connectivity of axons grown from neural stem cells transplanted into transected spinal cords [207]. The neural stem cells were grafted into transection sites in fibrin containing a cocktail of growth factors. Many thousands of axons from grafted neural stem cells grew out both rostrally and caudally, penetrating glial scars and areas of NG2 deposits, extending long distances, and making synapses with neurons to the brain and lower spinal cord. Host fibers entered the graft and made synapses with the neural stem cells. Interestingly, the rats showed some improvement in hind limb movement although they could not walk. Lu et al. subsequently showed similar results with induced pluripotent human stem cells [209].
In 2010, Liu et al. reported that conditional deletion of the phosphatase tensin (PTEN) homologue gene upregulated mammalian target of rapamycin (mTOR) and allowed remarkable regeneration of corticospinal tracts in mice [200]. Unlike the Lu studies where cells had been transplanted into the spinal cord and various manipulations might have altered the microenvironment of the spinal cord, the conditional deletion of PTEN occurred only in the motor cortex, the origin of the corticospinal axons that grew across the injury site. The axons grew across transection, hemisection, and crush injury sites without regard for the presence of myelin, glial scar, or inhibitory extracellular matrix glycoproteins. Recently, Du et al. from the Liu laboratory reported that PTEN deletion promotes regrowth of the corticospinal tract 1 year after injury in mice, suggesting that this treatment would be effective in chronic SCI [85]. However, despite many axons making synapses in neurons below the injury site, the mice did not recover walking. In 2014, Danilov et al. showed that conditional genetic deletion of PTEN enhances corticospinal regeneration and motor functional recovery after cervical SCI in mice [72].
The mechanism by which PTEN inhibits axonal growth is complicated. PTEN normally acts by converting phosphoinositol phosphate 3 (PIP3) to PIP2. Phosphoinositol 3 kinase (PI3K) converts PIP2 to PIP3, which in turn activates phosphoinositide-dependent protein kinase 1 and 2 (PDK1/2) to activate protein kinase B (PKB or Akt). Akt in turn inhibits tuberous sclerosis 1 and 2 (TSC1/2) to inhibit Ras homologue enriched in brain 1 (Rheb1). Rheb1 normally activates mTOR, which acts through several intracellular messengers to regulate protein synthesis and cell growth. Thus, in the absence of PTEN, PIP3 accumulates and activates Akt. Akt inhibits TSC1/2, which normally inhibits Rheb1, thereby disinhibiting Rheb1 to activate mTOR. This pathway can be influenced by many drugs. Thus, for example, lithium directly activates Akt, and this may be one of the mechanisms by which lithium stimulates spinal cord and peripheral nerve regeneration [56, 81, 105, 310, 355, 356]. Rapamycin is a potent inhibitor of mTOR. Lu et al. [207] found treating rats with rapamycin reduced axonal outgrowth in his experiments.
All By Itself
The currently accepted dogma is that the spinal cord cannot regenerate. This dogma arose from early studies of spinal cord transection models, which did more than just divide the spinal cord. First, because spinal cords are under tension, the stumps separate and a gap is almost invariably present after transection. Second, unless care is taken to preserve the anterior spinal artery, transections eliminate crucial blood supply and causes severe ischemia of spinal cord stumps and cystic necrosis at the injury site. Third, scientists seldom closed the dura after transection, allowing fibroblasts from surrounding tissues to migrate into the injury site, resulting in a fibrous scar. Axons will not grow readily across gaps, in necrotic and cystic spinal cord, or through fibrous scars. It is little wonder that most scientists did not think that the spinal cord can regenerate – but they did not consider that most SCIs do not involve cutting or transection of the spinal cord. A vast majority of human spinal cord injuries involve crush or contusion.
In 1995, Anghelescu et al. administered large doses of the glucocorticoid methylprednisolone or saline to rabbits after an experimental crush injury of the spinal cord [7]. Both treated and control groups of animals showed histological evidence of severe injury, including necrosis, vacuolization, cavitation, myelinic and axonal fragmentation, demyelination, and mesenchyme-glial scar reactions. However, in both treated and untreated groups, they observed large numbers of regenerated axons growing into the “dense scar of the injured cord.” Damaged fibers die back some distance from the injury site and grow back to the injury site. The growing axons were clearly not deterred by the inhibitory effects of myelin in the white matter, physical presence of glial scar, or deposits of chondroitin proteoglycans that surround the injury site. This study was largely ignored because it was published in an obscure journal and showed no difference due to treatment with methylprednisolone.
In 1997, the Multicenter Animal Spinal Cord Injury Study, involving eight leading SCI laboratories in the United States, reported that large numbers of spinal axons grow into the injury site of contused spinal cords, the type of injury that is the most common form of human SCI [19]. In 610 rat spinal cords contused with a 10-g weight dropped 12.5, 25.0, or 50.0 mm onto spinal cord exposed by T9–10 laminectomies, over 80 % of the spinal cords showed many axons growing into the injury site. In fact, the more severe the injury, the more axons grew into the injury site. Many were myelinated by Schwann cells entering from dorsal roots. A subsequent study by Hill et al. suggested that both ascending and descending spinal axons grew into and out of the injury site, including reticulospinal and corticospinal axons, despite the gliosis, myelin, and chondroitin proteoglycans around the injury site [132].
Since a majority of spinal cord injuries involve crush or contusions of the spinal cord, the observation of axon growth into the injury site and beyond in animal models of these injury mechanisms suggests that spontaneous regeneration may occur in many human SCI cases. This may explain why over 90 % of people will recover substantial function after even severe complete SCI, including independent walking [83]. Even after the so-called complete SCI, many people will recover 1–2 segmental levels of motor or sensory function [254, 345].
Perhaps it is time to reconsider the dogma that the spinal cord cannot regenerate and consider the possibility that some observed recovery in human spinal cord may be due to regrowth of long tracts across compressed or contused injury sites. The dogma was established over a century ago using models of SCI that are not clinically relevant. The weight of evidence in support of the ability of the spinal cord to regenerate is overwhelming.
Peripheral Nerve Effects on Spinal Cord Regeneration
Scientists have long known that prior injury of a peripheral nerve increases both rates of regeneration and numbers of regenerated fibers in the peripheral nerve. In 1983, Bisby et al. [26] noted that a prior crush injury of a sciatic nerve increased axon growth rates by 68 % after a second injury 7 days later to the nerve. In 1985, Bisby and Keen found that a conditioning injury stimulated small diameter unmyelinated axons containing substance P-like immunoreactivity to grow faster [27, 28]. Jenq et al. found that a conditioning injury not only increased the rate but also the number of regenerated fibers [153]. Bajrovic et al. showed that prior injuries enhanced the ability of axons to grow across acellular segments of crushed nerves [10]. These effects can be achieved by inflaming or compressing nerves [68–70]. Conditioning lesions resulted in faster growth initiation and initially faster axon growth, reduced long neurite extension, and fewer neuritic branches [184].
Do peripheral nerve lesions alter central axonal growth? In 1984, Oblinger and Lasek reported that peripheral axotomy did not affect regenerative properties of the central branch of DRG neurons [243]. Many investigators consequently thought that conditioning effects were limited to peripheral nerves. In 1989, Ebner et al. found that peripheral nerve damage facilitated functional innervation of embryonic brain grafts in adult sensory cortex [89]. In 1995, Woolf et al. reported that peripheral axotomy massively reorganized central terminals of primary afferents in the dorsal gray horn [347]. Then, in 1999, Neumann and Woolf showed that peripheral nerve lesions stimulated central axonal growth of DRGs across dorsal column lesions made 2 weeks later [236]. In the same year, Chong et al. cut and anastomosed L4, L5, and L6 dorsal roots of rats and found that injuring the ipsilateral sciatic nerve greatly increased the number of dorsal root axons penetrating and growing in the dorsal column [59]. In 2002, Neumann et al. showed that intraganglionic administration of cAMP induced regeneration of sensory axons in injured spinal cord [237]. In 2005, Neumann et al. showed that peripheral nerve injury after SCI stimulates central regeneration [238].
Many investigators have studied the mechanisms by which conditioning peripheral nerve injuries enhance DRG neurons to regenerate. In 1999, Lazar et al. found that peripheral nerve injuries modulated macrophage and microglial responses to axonal injury and proposed that these responses may contribute to the regeneration in the spinal cord [187]. Delcroix et al. showed that peripheral nerve injury upregulated activating transcription factor 2 (ATF-2) in nociceptive DRG neurons and may be responsible for neuropathic pain [78]. In 2006, Seijffers et al. found that ATF-3 promotes neurite outgrowth of DRG neurons and that peripheral nerve lesions but not central lesions induced ATF-3 expression in DRG neurons and subsequently showed that ATF-3 expression increased the intrinsic growth state of DRG neurons [297, 298]. In 2005, Qiu et al. showed that sciatic nerve transection caused transient phosphorylation and activation of the transcription factor signal transducer and activator of transcription 3 (STAT3) in DRG neurons [260]. Blocking STAT3 prevented the effects of the conditioning lesion. Interestingly, we recently showed that lithium directly blocks STAT3 in neural stem cells and suppresses astrogliogenesis and also reduces severe neuropathic pain in patients with chronic SCI [354, 371].
Neuronal cAMP levels regulate growth cone behavior. In 1997, Ming et al. found that Netrin-1, a well-known chemoattractant for neurons, guides growth cones by increasing cAMP [229, 305]. The growth cones of Xenopus spinal neurons showed chemorepulsive responses in the presence of a competitive analog of cAMP or an inhibitor of protein kinase A (PKA). Song et al. showed that changing cAMP switched turning directions of nerve growth cones and that a gradient of brain-derived neurotrophic factor (BDNF) triggers attraction of the growth cones to the higher BDNF concentrations but that the presence of a competitive analog to cAMP or inhibition of PKA induces repulsion [305]. In 2003, Qiao et al. reported that PKA activation inhibits RhoA, the protein that is known to mediate inhibition of axonal growth [259]. Increasing neuronal cAMP levels blocks inhibition of axonal regeneration by MAG and myelin [46, 47].
The Importance of Rehabilitation
Despite rivers of axons crossing the injury site and making synaptic connections above and below the injury site in the studies by Liu et al. and Lu et al., animals recovered relatively little function after complete transections [85, 200, 206, 207]. Interestingly, none of these studies did any rehabilitation of the rats. The reason for the lack of recovery is evident. The rats do not know how to use any of the new pathways that have regenerated. Without activity, synapses do not consolidate. Much evidence suggests that motor recovery does not occur without exercise [94, 274]. Rehabilitation was essential for recovery.
In 2009, Garcia-Alias et al. found that ChABC treatment opens a window of opportunity for task-specific rehabilitation after SCI [107]. Rats received a C4 dorsal funiculus, followed by ChABC or penicillinase control therapy and different types of rehabilitation. The rats recovered functions that are specific for the rehabilitation they received and rehabilitation for certain function may be deleterious for other function. For example, locomotor training resulted in locomotor recovery and worse skilled forelimb reach behaviors. In 2014, Zhang et al. showed that combining treadmill training with semaphorin3A inhibition helped rewire central pattern generators and improved locomotion in rats [366]. In 2015, Ishikawa et al. showed that a combination of keratin sulfate digestion or ChABC and rehabilitation promoted anatomical plasticity in injured rat spinal cords [150].
ChABC increases plasticity of the motor cortex, respiratory motor system, and lumbosacral spinal cord after SCI [96, 108, 150, 224, 245, 246, 335]. Likewise, blockade or deletion of Nogo and NGR1 increases plasticity [38, 295, 301, 306, 318]. For example, Zenmar et al. reported that anti-Nogo or anti-NgR1 antibodies increased long-term potentiation of pyramidal cells induced by stimulating horizontal fibers [364]. These treatments may extend the period of time during which rehabilitation improves functional recovery.
Clinical experience also suggests that locomotor training early after SCI improves recovery [20, 177, 370]. Walking activates multisegmental monosynaptic responses [65, 87, 129, 175, 176]. The functionally isolated spinal cord is plastic and has the capacity to generate locomotor patterns with appropriate afferent input, to undergo periods of spontaneous activity, and to make decisions to generate successful postural and locomotor tasks [127, 219, 271].
Electrical stimulation provides activity needed for synaptic consolidation [358]. In 2007, Brus-Ramer et al. reported that electrical stimulation of spared corticospinal axons augments ipsilateral spinal motor circuits after injury [40]. This effect is not mediated by the corpus callosum but through latent or newly sprouted connections by the spared motor cortex [41]. In 2010, Carmel et al. showed chronic electrical stimulation of the spared motor corticospinal tract after spinal cord hemisection induced outgrowth of spared axons into spinal cord below the hemisection to restore skilled paw placement on the impaired side [51–54].
Epidural stimulation of the spinal cord has recently become popular because of reports that such stimulation restores voluntary, standing, and locomotor function [6, 90, 128, 278]. Animal studies show that such epidural stimulation can not only improve locomotion but improves urethral relaxation during voiding and reduces hyperalgesia and neuropathic pain [3, 276, 325]. Several investigators have empirically developed criteria for optimal electrode placement and stimulation parameters for rats, monkeys, and humans [4, 278, 299]. Epidural and functional electrical stimulation does not replace rehabilitation. Electrical stimulation may increase the rate and extent of functional recovery associated with intensive directed rehabilitation.
The data strongly affirms the old maxim, “use it or lose it.” Brain and spinal cord cannot afford to maintain unused circuits. Regenerated connections, if not used, will fade and disconnect. Exercise and directed rehabilitation is essential for functional recovery. We must not judge regeneration without rehabilitation harshly.
Peripheral Nerve Regeneration
Peripheral nerve injury is increasing in both incidence and severity, as populations increase together with concomitant increases in traffic and industrial accidents, violence, and battlefield injuries. It has been estimated that global nerve repair and regeneration costs will increase from $4.5 billion in 2013 to $7.8 billion in 2018 [213]. The current gold standard of care involves autologous repairs: direct repair (gaps of ~0–1 cm), nerve autografts (replacement nerves from elsewhere in the patient, usually the sensory, sural nerve; for gaps of ~1–10 cm), or vascularized nerve autografts (for gaps ~10 + cm) [25, 62, 316].
Autologous Repairs and Timing
In contrast to the belief that spinal cords cannot regenerate, scientists and clinicians have long agreed that peripheral nerves will regrow, make synaptic connections with muscles and sensory organs, and are remyelinated by Schwann cells. Recovery takes place over many months and is consistent with distance and slow rate of axonal growth (~1 mm/d). Thus, unlike the field of spinal cord regeneration, the field of peripheral nerve regeneration has not been stymied by the dogma that regeneration cannot occur. In clinical practice, a vast majority (90 %) of patients with peripheral nerve injuries are treated by primary nerve repair, elective delayed nerve repair, or primary surgical exploration [216].
Animal studies suggested that the critical period for peripheral nerve repair is 1–2 months. In 1988, Bolesta et al. reported that immediate repair of rabbit sciatic nerve had the best results, but 1–6 months delay resulted in 25–50 % less function [29]. In 1989, Gattuso et al. compared immediate and delayed repair of peripheral nerves using freeze-thawed autologous skeletal muscle grafts in rat at 3, 5, 7, 14, 28, and 56 days after transection [110]. A delay of 56 days was found to be incompatible with useful recovery, but myelinated nerve fiber number and diameters of nerves repaired in 3–28 days did not differ significantly from immediate repaired nerves. In 1994, Baranowski et al. compared DRG neurons in rats that were repaired immediately or 3 months after sural nerve transection [15]. The number of surviving DRG neurons was 60 % after both immediate and delayed repair, suggesting that loss of sensory neurons is not the reason. In 1995, Lawson and Glasby used freeze-thawed muscle grafts to repair 3-cm gaps in median nerves in sheep. Five were repaired immediately (A) and 5 after 4 weeks. Peak nerve conduction velocities were significantly slower in repaired nerves; mean fiber diameters were 5.06, 3.90, and 8.58 μm in the immediate, delayed, and control groups. Short delays of 3 days have little effect [253]. In 2013, Wu et al. standardized a rat model of delayed sciatic nerve repair, showing that the compound action potentials were worse only after 6-, 8-, and 12-week delays [349].
Clinical experience confirms poor results in delayed nerve repairs [135, 144, 174]. In 2010, Mohseni et al. in Iran reported that early primary repairs have the best results with all 60 patients showing some recovery and 25/65 (38 %) having excellent results, compared to poor recovery and 7/25 (28 %) failures with delayed primary repair and 5/15 (33 %) failures after delayed nerve graft [231]. In 2014, George and Boyce reviewed 28 studies (1577) of peroneal nerve repairs [111]. Good results were obtained in 45 % of cases: 80 % for neurolysis, 37 % for primary repair, and 36 % for nerve graft. Excluding neurolysis, good outcomes were obtained in 44 % of repairs performed within 6 months and 12 % of repairs done after 12 months. These results do not seem better than recovery from SCI.
Many investigators focused on changes in the distal stump or end organs. In 1985, Pellegrino and Spencer pointed out that Schwann cells undergo atrophy in distal stumps that have been denervated for long periods [250]. In 2005, Midha et al. attempted to protect the distal stump by cross-suturing the quadriceps motor nerve (motor) or re-suturing of the saphenous nerve (sensory) for 8 weeks [225]. More myelinated axons innervated motor- than sensory-protected or unprotected nerve. They concluded that even a 2-month denervation of distal nerve is deleterious to regeneration and that protecting the distal stump with another nerve improves subsequent reinnervation and regeneration. Ronkko et al. showed no difference in the capacity of the distal stump to receive growing axons after 2- or 6-month denervation in rats [270]. In 2013, Jonsson et al. in Sweden compared immediate (0)-, 1-, 3-, or 6-month delays in nerve graft repair of transected sciatic nerves [159]. The number of regenerating motoneurons and myelinated axons in the distal nerve stump declined dramatically in the 3- and 6-month groups with greater muscle atrophy and neuromuscular junction changes. Key changes of muscles include fragmentation of the neuromuscular junction [350]. Electrical stimulation accelerates peripheral nerve regeneration up to 1 month after injury but not in nerves that were repaired more than a month after injury, possibly due to fibrosis in the distal stump [92, 124, 340, 351].
Nerve Guidance Conduits and Neurotrophic Devices
Despite being the current gold standard, autografts have significant drawbacks, including the surgical procedure to harvest a donor nerve, with its associated morbidity; limited options for matching length and size of donor nerves to the injured nerve; and sensory-mixed mismatching, i.e., the mismatches that results from donor nerves typically being sensory, when most of the defects they must repair are typically mixed (motor and sensory). Therefore, many clinicians have sought alternatives as far back as 1880 [149]. Kaplan et al. reported that this effort has accelerated in the past five decades, with published studies on the subject almost doubling over the past two decades [167]. Although solutions for bridging short nerve gaps have been somewhat effective, repairs of long nerve gaps (5–30+ cm) remain less effective and are a key research challenge.
Nerve guidance conduits (NGCs) are an alternative to autograft repairs. These conduits generally consist of a tube filled with axon growth supporting material and neurotrophic factors. Kaplan et al. and Windebank’s group have both noted that testing of NGCs has been limited mostly to the rat sciatic nerve injury model [8, 167]. As Kaplan et al. pointed out: “(1) The preponderance of nerve regeneration data is now in a single species, which is likely to skew treatment outcomes and lead to inappropriate evaluation of risks and benefits. (2) The rat is a particularly poor model for the repair of human critical gap defects due to both its small size and its species-specific neurobiological regenerative profile: The real mismatch is that the rat model is used to test NGCs over 1–1.5 cm gaps, while they are ultimately supposed to work clinically in 5–30 cm gaps. (3) Translation from rat to human has proven unreliable for nerve regeneration, as for many other applications.”
Clinicians currently used several NGCs to repair nerves, including decellularized allografts (decellularized nerves from human cadavers, e.g., Avance® made by AxoGen, Alachua, FL), vein and other biologic conduits (type I collagen, e.g., Neuragen® made by Integra Life Sciences, Plainsboro, NJ), and synthetic conduits (such as woven polyglycolic acid, e.g., Neurotube® made by Synovis Micro Companies Alliance, Birmingham, AL) [313]. Allografts or synthetic conduits avoid the morbidity of harvesting a donor nerve but are less effective in supporting regeneration. Disadvantages of vein conduits and other biologic NGCs include collapsing, kinking, lack of neurotrophic support, and promotion of scarring (e.g., some components such as collagen promote fibroblast activity). Synthetic NGCs can be designed to avoid the disadvantages of biologic NGCs, but currently synthetic NGCs cannot match the performance of autografts and allografts and offer only limited functional recovery.
To date, few synthetic NGCs have achieved US Food and Drug Administration (FDA) or Conformité Européenne (CE) approvals for clinical use (only 4 by 2014) and almost exclusively for repair of short gaps (≤4 cm; one ≤6.35 cm) [71, 248]. New NGCs must address two major issues. First, chemical and mechanical properties of the conduit wall must be optimized to facilitate handling, to be suturable, to have appropriate stiffness and flexibility, and to resist compression and kinking for longer nerve gap repairs and for crossing moving joints of the finger, elbow, shoulder, or knee. Second, effective biological enhancement strategies must be incorporated into synthetic NGCs, to create a biological milieu within the conduit that supports and directs nerve growth.
Each of the three main components of the NGC, i.e., the wall, the filler, and bioactive moieties, must be optimized. This requires testing variants of each alone and in combinations, to identify designs that will support nerve regeneration while inhibiting scar infiltration, be kink and compression resistant while offering superior handling and suturability, and will allow nutrient and waste exchange while retarding the ingress of fibrous tissue. In vivo studies are critical for preclinical testing. Most academic laboratories rely on rat sciatic nerve injury models to test NGCs. The sizes of rats limit the nerve gap to 1–2 cm and the sciatic nerve injury model, so commonly used does not expose NGCs to significant mechanical forces or bending. Furthermore, rats have a strong regenerative potential compared to human patients, who are often old and suffer from diseases that compromise healing and regeneration, such as diabetes.
Variations of neuroregenerative potential between species further complicate interpretation of preclinical results [282]. A “critical nerve gap” is a nerve gap over which no recovery will occur without some form of nerve grafting or bridging [71, 316]. The critical nerve gap is ~1.5 cm for rats, ~3 cm for rabbits, and ~4 cm for pigs and humans. These differences exist despite the fact that longitudinal axonal growth rates are similar across most species, at approximately 1 mm/d (potentially due to the primitive and phylogenetically common ancestry of the neurite lengthening machinery involved) [122]. This phenomenon may be explained by differing rates of fibrin degradation across species: Early on in the nerve repair process, an acellular fibrin-extracellular matrix cable is laid down between the proximal and distal stumps [21, 71, 134, 342]. In rats, this occurs within 1 week of repair and is followed by cellular infiltration. Schwann cells, endothelial cells, and fibroblasts migrate into the cable from both the proximal and distal nerve stumps. The Schwann cells proliferate and align to form parallel cables (the “glial bands of Büngner”), which provide neurotrophic and structural support for axonal regeneration. The fibrin cable is a critical component of this process and, due to variable thicknesses and degradation rates across species, is believed to define the maximum gap length over which healing can occur. Its degradation time is about 2 weeks in the rat, and up to 4 weeks in the human, leading to critical nerve gaps of ~1.5 cm in a rat sciatic nerve model and ~4 cm in humans [163, 251].
Into the Future
Why just restore function? Why not exceed normal function? These questions generate quandaries of such proportions that they are fit for an entire ethical textbook and certainly cannot be done justice here. We leave the reader to consider for themselves the risks and benefits of what is now already achievable, and the future possibilities, some examples of which we explore here. Author Kaplan proposes that we are nearing and, in some cases, are already capable of achieving, what he coins “Nervous System 2.0” (NS2.0) and the “Neural Enhancement Divide” (NED) – which is that inflection point where instead of restoring the nervous system, we begin to improve on it!
NED: The End or the Beginning?
Ethical issues abound when we can make some individuals better than “normal.” Arguably, the first example ever is that of the bilateral lower limb amputee runner Oscar Pistorius. In the 2008 Olympics, Oscar’s team lobbied furiously to allow him to run in the Olympics rather than in the Para-Olympics but to no avail as it was widely considered unfair to Pistorius as he would be disadvantaged. That year, at the Para-Olympics, he won his races spectacularly. In 2012, after much lobbying once again, the team managed to gain entry to the Olympics, and Oscar went on to beat all the “able-bodied” athletes, running on 2 C-legs. This time, there was an outcry that he might have been unfairly advantaged by his prosthetics.
Although we have not reached the NED yet, the time is coming for our nerve regenerative and restorative capabilities to do so. Is it reasonable to add infrared or X-ray vision to retinal implants? Is it fair to literally provide “superhuman” strength through implantable extra muscles or mechanical actuators that may be implanted or at least part of wearable exoskeletons? Is it reasonable to provide unlimited computing power through visual system digital overlays or interfaces directly with the brain? The list is inexhaustible.
Recently, much discussion has revolved around the relatively easy and cheap use of the CRISPR/Cas system for modifying our genetic code [130, 155, 188]. First described by Jinek et al. in mid-2012, the publication of this technique – that offers such great promise – still precedes any adequate ethical or legal framework to contain it [155]. Is it safe or ethical for researchers to be able to readily change the DNA of almost any organism? How will this impact our futures and, existentially, the evolution of all species? Two sides to this argument have arisen. One school believes that we should use the utmost caution and aggressively control genetic modifications, while at the other extreme, there are those who believe that our acquiring the capability to modify genetic code, and modifying our own code, is in fact part of evolution itself!
Whichever school of thought you subscribe to, the NED is fast approaching and it is inevitable.
Nervous System 2.0
Regeneration and/or restoration of function beyond the NED, to create a super/augmented/enhanced NS2.0, will no doubt be achieved through both biologic mechanisms (as discussed above, and many of which are yet to be elucidated still) and through – or in combination with – neural prosthetic mechanisms.
Loeb defines neural prosthetics as “the subset of neural control concerned with replacing and repairing neural function via electronic interfaces” [202]. In his fascinating chapter, “We Made the Deaf Hear. Now What?”, he describes how when cochlear implants were first being developed (circa 1975), many expert auditory neurophysiologists believed that a functional auditory prosthesis could not be achieved [201]. The biophysics of electrically excitable tissues had been well established already for about 50 years, and clinical experiments had already demonstrated that sounds could be perceived through electrical stimulation. Their concerns were, instead, based on their beliefs of how the CNS might process and perceive the wide variety of temporospatial patterns generated by the electrical activity of so many auditory neurons. Human hearing covers frequencies from 20 to 20,000 Hz (cycles/s). It was believed by some that computing, interpreting, and stimulating signals that covered approximately 20,000 frequency possibilities, each with their own varying temporal, spatial, and intensity profiles, were an impossible task, which was furthermore, significantly compounded by the varied perceptions of sounds by different patient types (e.g., previously hearing vs. congenitally deaf).
On the other hand, around the same time, researchers who were starting to work on retinal implants generally felt confident that success could be achieved relatively more easily, because of our ability to gather useful information from images containing as little as 4 × 4 = 16 pixels (vs. 20,000 frequencies for hearing) [66].
So for many years, it was commonly believed that retinal implants would be more easily achievable than cochlear implants, but in fact the exact opposite has proven true: While auditory neurophysiologists were struggling with the best ways to approach cochlear nerve stimulation, in an unrelated but serendipitous effort, AT&T were struggling with the best ways to carry wide frequency bands of data over copper telephone wires. The work they did showed clearly that we could understand conversation with as few as just eight bands of averaged frequencies across the speech region of the audible frequency spectrum (20–6000 Hz).
Suddenly cochlear implants with just eight electrodes could prove extremely useful. It also taught us the importance of miniaturizing the cochlear implant electrodes sufficiently to achieve the lower frequencies required, as these are tonotopically mapped along the basilar membrane toward its narrowest tip in the cochlea’s “snail shell.” Today, cochlear implants with 16–24 electrodes are becoming the standard, offering even music appreciation! While, on the other hand, visual prosthesis researchers are still working to elicit small and reproducible phosphenes, and attempting to achieve stable long-term visual percepts. Much of the recent research is still conducted using arrays with very limited numbers of electrodes, e.g., the A16 (4 × 4 electrodes) and Argus II (6 × 10 electrodes) by Second Sight Medical Products Inc. (Sylmar, California, USA) [66] and a 9 × 9 research array proposed by Monash University [192].
These examples of two neural prosthetics highlight two important points for a future NS2.0. Firstly, unexpected developments in seemingly unrelated fields can hasten the understanding and development in neural prosthetics, driving the NED and ultimately making NS2.0 capabilities possible that we have yet to envision. Secondly, unexpected and poorly understood neurophysiological phenomena can retard the NED, prohibiting us from even just restoring normal function to our nervous system 1.0. Clearly, the NED will be achieved for varying neural functions throughout the future of humanity, some in coming decades, and others over many centuries to come, as Loeb describes in his chapter, “Neuroprosthetic Interfaces – The Reality Behind Bionics and Cyborgs” [202].
NS2.0 Possibilities
In Michio Kaku’s enlightening book, Physics of the Impossible: A Scientific Exploration into the World of Phasers, Force Fields, Teleportation, and Time Travel, Kaku explores physical possibilities and categorizes them into three classes of impossibilities: Class I Impossibilities are impossible today but might be possible within this century; Class II Impossibilities are those that sit at the very edge of our understanding and might be realized on a scale of millennia, if at all; and Class III Impossibilities are technologies that violate our known laws of physics and would involve a fundamental shift in these laws if they ever proved possible [162].
Taking Kaku’s lead, Kaplan would like to propose considering NS2.0 possibilities in a similar fashion, by categorizing them into three classes of possibilities (rather than impossibilities). As our understanding of neurobiology is progressing on a different timescale to the laws of physics, I would propose here that Class I Possibilities are those that are almost possible today and might be possible within the next three decades; Class II Possibilities are those that still sit at the edge of our understanding and might be realized beyond three decades to centuries; Class III Possibilities are technologies that could have great utility, but on which no substantial research achievements have yet been made.
The following are just some examples of current technologies that may ultimately lead to future NS2.0 technologies.
Class I Possibilities (Within 30 Years)
Advanced Tissue Regeneration
We have discussed in detail above the many advances that are being researched in terms of spinal cord and peripheral nerve regeneration from a biological point of view. Tapping into the inherent regenerative capabilities of the human body will continue to yield remarkable advances over the coming decades, particularly together with the influence of other emerging fields such as DREADDs (Designer Receptors Exclusively Activated by Designer Drugs) [58, 249]. While open to debate, we believe that these Class I Possibilities will vastly improve the degrees of partial recovery that we are currently seeing in both the CNS and the PNS.
However, true and full regeneration of the brain, spinal cord, and peripheral nerves remain Class II Possibilities. Loeb refers to the opportunity for “Augmented Plastic Recovery,” based on the relatively recent acceptance in neuroscience that adult brains are in fact capable of substantial reorganization and of regenerating some neurons [156, 202, 223]. While traditionally patients with strokes, traumatic brain injuries, and SCIs have been taught to live with their disabilities, the emphasis over the past two decades has grown to include recovery of function. Intense exercise and training programs are now part of standard physical therapy treatments for these patients, often enhanced by robotic aids that adjust the levels of support and challenge the patient receives in real time [326]. Loeb explains that electrical stimulation of muscle and nerves produces patterned electrical activity that projects back to the spinal cord and brain, where it may provide neurotrophic effects.
Embedded Sensory-Motor Activity for Prosthetic Limbs
Nghiem et al. have recently reviewed methods of providing a sense of touch for prosthetic hands [239]. One such technique that shows great promise for not only sensory activity but for prosthetic motor activity as well is “targeted reinnervation,” as originally described by Kuiken and Dumanian et al. in 2004 [179, 180, 202]. This involves rerouting the major nerve trunks of the upper limb (radial, median, and ulnar) into the anterior chest wall during or after an amputation, instead of burying them in stump muscles as is traditionally done to minimize the risks of neuroma and/or phantom limb pains. In the anterior chest wall, these nerves are anastomosed to the nerves to the chest wall muscles (pectoralis major and serratus anterior) and to the nerves that supply sensation to the overlying skin. Now, after healing, the reinnervated chest wall targets are mapped out for motor function via electromyography (EMG) recording patterns over the chest wall during attempted upper limb movements and for sensory function by determining upper limb sensations experienced during electrical stimulation of the chest skin via surface or wirelessly implanted electrodes at a grid of sites over the chest wall. Within an afternoon, this mapping procedure provides a data set that can drive and receive sensory input from a prosthetic device, without any patient learning required at all. This paradigm shift in how we control and sense prosthetic limbs has bypassed centuries of work on controlling prosthetics through patient or machine-learning techniques!
Another exciting development is the robotic finger pulp developed by Loeb et al. [339]. This award-winning biomimetic “finger tip” comprises several electrodes along a rigid “phalynx” of plastic, embedded in an electrically conductive gel surrounded by a silicon “skin.” Small changes in the amount of gel between the electrodes can be measured electrically with such sensitivity that tiny vibrations of a slipping cup, for example, can be detected and adjusted for, so that a robotic hand with these fingers can pick up a Styrofoam cup without crushing it or dropping it or determine if a piece of fruit is ripe without bruising it. Clearly, these techniques are still approaching restoration of our nervous system’s function, but over the coming decades, it could be feasible to insert a cam into the motor pathway so large movements at the chest level can be converted into minute movements of the arm or vice versa; that tremors may be removed if necessary, etc. Similarly, one could now “sense” how hot a kiln is inside, or pick up a giant metal beam, or …
Superhuman Strength
Through implantable extra muscles or mechanical actuators that may be implanted or at least part of wearable exoskeletons or through robotic arms as discussed above, superhuman strength may certainly become a reality. Surgical feasibility for implanting an additional muscle has already occurred, and exoskeletons with EMG pickup and electrical stimulation capabilities exist [60, 329]. Refining these technologies is a matter of time.
Unlimited Computing Power Through Visual System Digital Overlays
One example of this possibility may be the combination of a Google Glass-type computer with a retinal implant of sufficient resolution to scroll a text overlay initially and to provide a high-resolution detailed virtual environment overlay ultimately.
Floating Light-Activated Microelectrical Stimulators (FLAMES)
Neural stimulation and recording are usually performed using fine wire microelectrodes. Not only are these inserted traumatically, causing damage to the spinal cord, brain, or peripheral nerves, but their rigidity, relatively large size (on the order of 100s of microns), and fixed, tethered nature lead to scarring, with increasing thresholds for stimulation and decreasing signal-to-noise ratio for recording. Furthermore, the fine cables that connect to the driving electronics break easily. Together, these events severely limit the functional lifespan of implantable electrodes, in some cases to just weeks to months. FLAMES are a new technology that may solve some of these issues by providing wireless neural stimulation in submillimeter devices of ~150 μm in thickness [1]. Once implanted, a near-infrared laser beam transfers energy to the devices through the tissues to wirelessly activate the devices via their photodiodes and generate stimulus current. To date, prototype testing in a rat model has demonstrated successful intraspinal stimulation resulting in forelimb movement.
Fetal Pacemaker
Currently if a fetus requires a pacemaker, in utero surgery is performed at great risk. Loeb et al. have developed a prototype injectable microstimulator to avoid the associated risks and have tested it in vivo [14, 203]. This device is injected through the mother’s abdomen and the soft cartilaginous sternum of the fetus, under ultrasound guidance, and the distal corkscrew electrode is then screwed into the myocardium where it remains until a definitive pacemaker can be implanted after birth. It is powered by a small battery with or without inductive charging if needed. Over the coming decades, this is likely to translate into clinical use, and the idea could potentially be applied to other applications, such as in utero diaphragm pacing or neuromuscular electrical stimulation (NMES) for cerebral palsy, as examples.
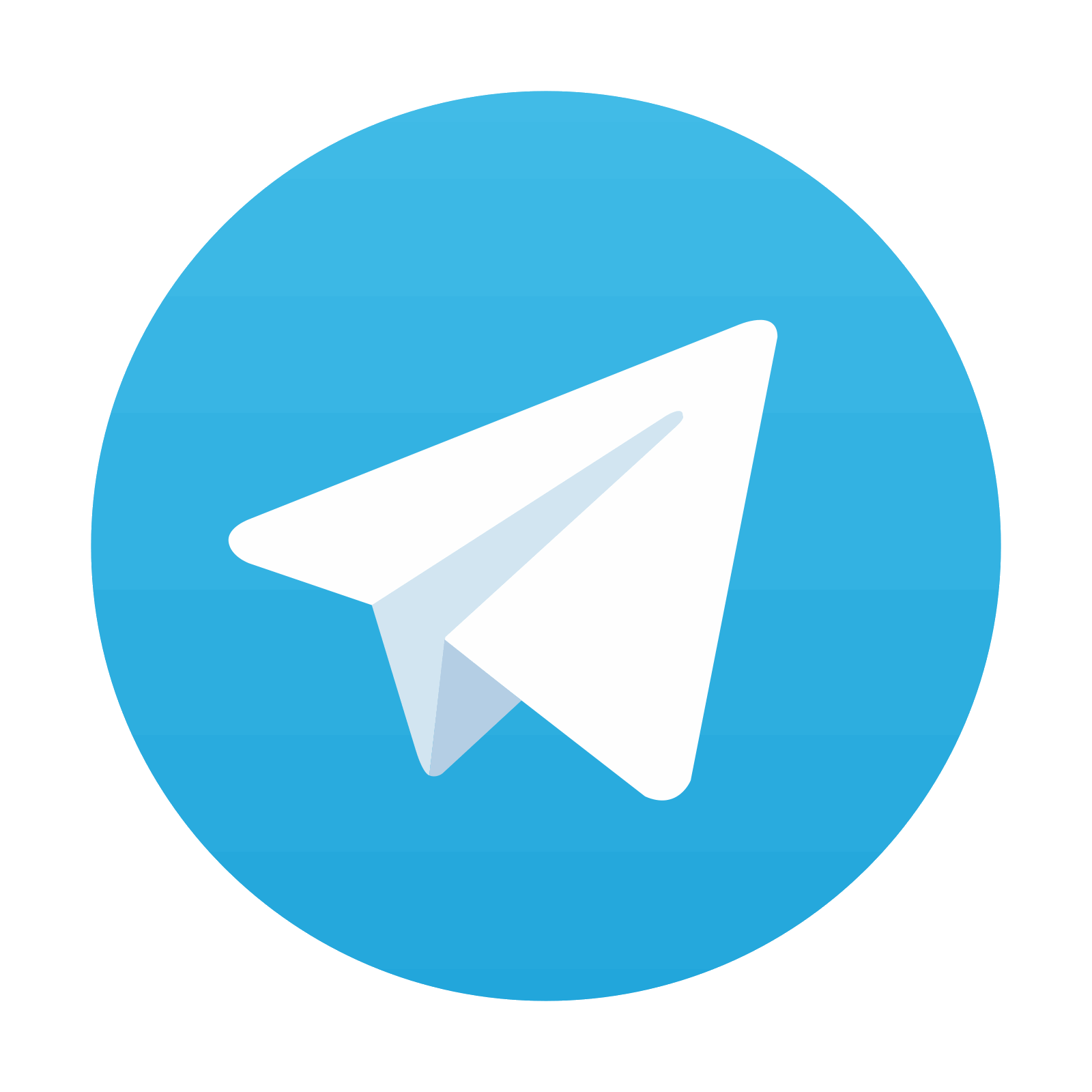
Stay updated, free articles. Join our Telegram channel
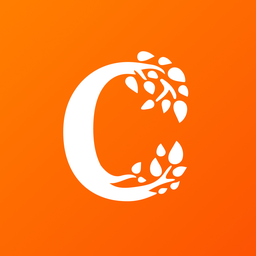
Full access? Get Clinical Tree
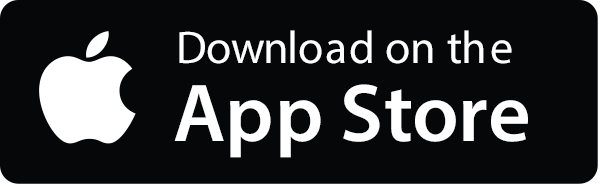
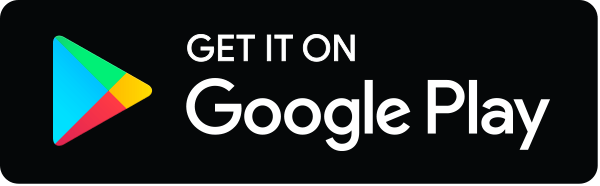
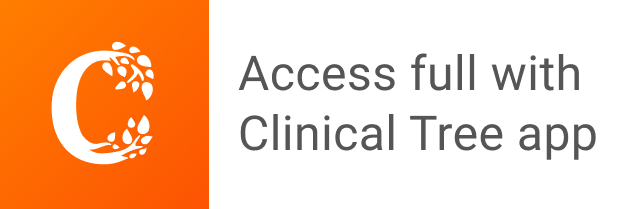