1 Soft tissue distress
Somatic dysfunction
The musculoskeletal system – sometimes referred to as the locomotor system – is the means whereby we act out and express our human existence – ‘The primary machinery of life’ is what one of osteopathy’s greatest researchers, Irwin Korr (1970), called it. While, medically speaking, the musculoskeletal system may lack the glamour and fascination of vital organs and systems, the fact is that the cardiovascular and neuroendocrine and digestive (and other) systems and organs exist only to service this great biomechanical structure through which we live and function. It is by means of our musculoskeletal system (not our kidneys or livers) that we perform tasks, play games, make love, impart treatment, perform on musical instruments, paint and, in these and a multitude of other ways, interact with one another and the planet.
The musculoskeletal system – comprising mainly bones, muscle and connective tissue structures, such as ligaments, gives shape and stability, and allows movement, to the human body, while also protecting the vital organs (Mooar 2007).
The term ‘somatic dysfunction’ offers an accurate and a comprehensive term to describe all lesions of the musculoskeletal system – osseous and soft tissue (Licciardone 2005).
Sometimes somatic dysfunction is the primary cause of pain, however sometimes it generates reflexive symptoms, or may act as a perpetuating feature. Palpation may provide clues to the true nature of an injury (Stanton 1996). A process of assessment and examinations may help to identify structural factors associated with pain and dysfunction, often associated with repeated injury or cumulative microtrauma involving postural, habitual or occupational ergonomic stresses.
Identifying sources of pain
Different dysfunctional tissues produce different qualities of symptoms (Kuchera 2005). For example: sclerotomal tissues (skeletal, arthrodial, and ligamentous generators) typically relate to dull, aching pain – which may be experienced some distance from the actual source; myotomal (muscle) pain is also poorly localized, possibly also at a distance from the generating site. Trigger point activity in hypertonic tissues might be involved. The pain is typically described as involving stiffness, aching and sometimes having a cramp-like quality. Note: Examples of sclerotomes and myotomes are illustrated in Chapter 5, while the palpatory characteristics (such as tissue texture changes) of dysfunctional tissues that generate pain locally, or at a distance, are to be found in Chapter 3.
The objectives, if not the methods we are discussing, are not new. Carl McConnell, a major force in early 20th century osteopathy, discussed the soft tissues as follows (McConnell 1962):
Coherent and incoherent patterns
In Chapter 3 we will examine one of the major causes of somatic pain and dysfunction, myofascial trigger points, and the causes of this widespread phenomenon. It will then become clear that, while many forms of (referred) pain follow predictable and neurologically coherent pathways, there also exist patterns of pain and dysfunction that do not.
Reporting stations
The reporting mechanisms in the soft tissues and joints (Travell & Simons 1983, 1992, Wall & Melzack 1991) may be thought of as providing answers to a number of basic questions that the central nervous system (CNS) requires answering.
These questions were expressed by Keith Buzzell (1970) as follows: ‘What is happening in the peripheral machinery with respect to three questions? What is the present position? If there is motion, where is it taking us? And, third, how fast is it taking us there?’
The various neural reporting organs provide a constant information feedback to the CNS and higher centres as to the current state of tone, tension, movement, etc. of the tissues housing them. Such sensory information can be modulated and modified both by the influence of the mind, and by changes in blood chemistry, to which the sympathetic nervous system is sensitive. A variety of inputs of information will give the answers to these important questions so that the body can provide appropriate responses to the demands and adaptations constantly called for by varying situations. Some important structures involved in this internal information highway are summarized in Box 1.1.
Box 1.1 Reporting stations
Muscle spindle
The proprioceptive role of muscles of the suboccipital region is directly related to the number of spindles per gram of muscle. There are an average of 36 spindles per gram in some of the suboccipital muscles, such as rectus capitis posterior minor, and 30.5 spindles per gram in rectus capitis posterior major, compared, for example, with 7.6 spindles per gram in splenius capitis and just 0.8 spindles per gram in gluteus maximus (Peck et al 1984). McPartland & Brodeur (1999) suggest that ‘The high density of muscle spindles found in the RCPM muscles suggests a value … [which] lies not in their motor function, but in their role as “proprioceptive monitors” of the cervical spine and head.’
Buzzell (1970) describes the neural connections with the CNS thus:
In contrast, the secondary fibres have various synapses in their central connection which can be traced to higher cortical centres. Conscious activity may, therefore, provide a modifying influence, via these structures, on muscle tone. The activities of the spindle appear to provide information regarding length, velocity of contraction and changes in velocity. How long is the muscle? How quickly is it changing length? And what is happening to this rate of change of length? (Gray 1977).
There are various ways of ‘manipulating’ the neural reporting stations to produce physiological modifications in soft tissues – notably of the Golgi tendon organ in muscle energy techniques (METs) and of the spindle in various positional release (PR) techniques, such as strain/counterstrain (SCS) (Jones 1980, Stiles 1984).
Effect of contradictory information
Korr’s words regarding the nature of the information that these, and other, reporting stations are providing to the CNS are worth recording (Korr 1976). He reminds us:
Contradictory gibberish?
When somatosensory, vestibular or visual afferent systems provide conflicting information, a variety of symptoms may result. Somatosensory afferent systems depend on coherent input, from the soles of the feet, the neck and lumbar spine (Gagey 1986).
Neural ‘cross-talk’
Korr (1976) discussed a variety of insults that could result in increased neural excitability: the triggering of a barrage of supernumerary impulses, to and from the cord, and also what he terms ‘cross-talk’, in which axons may overload and pass impulses to one another directly; muscle contraction disturbances, vasomotion, pain impulses, reflex mechanisms, disturbances in sympathetic activity, all may result from such activity, due to what might be relatively slight tissue changes, for example in the intervertebral foramina.
He summarized thus (Korr 1976):
Mechanisms that alter proprioception
• Ischaemic or inflammatory events at the receptor site may produce diminished proprioceptive sensitivity due to metabolic byproduct build-up stimulating groups III and IV, mainly pain afferents (this also occurs in muscle fatigue).
• Physical trauma can directly affect receptor axons (articular receptors, muscle spindles and their innervations).
• In direct trauma to muscle, spindle damage can lead to denervation and atrophy (e.g. following whiplash) (Hallgren et al 1993).
• Structural changes in parent tissue lead to atrophy and loss of sensitivity in detecting movement, plus altered firing rate (e.g. during stretching).
• Loss of muscle force (and possibly wasting) may result when a reduced afferent pattern leads to central reflexogenic inhibition of motor neurons supplying affected muscle.
• Psychomotor influences (e.g. feeling of insecurity) can alter patterns of muscle recruitment at the local level, and may result in disuse muscle weakness.
Trophic neural influences
Setting aside for the moment the obviously important feature of nerves, and their message-carrying functions, we need to consider the less understood role they play in transporting substances – proteins, phospholipids, glycoproteins, neurotransmitters, enzymes, mitochondria and more (Canals et al 2004).
Transportation takes place, at a rate of anything from 1 mm/24 h to several hundred millimetres per 24 h, depending on what is being transported and the presence, or absence, of interfering factors. Movement occurs in both directions along nerves, with retrograde (returning from the target tissues towards the CNS) transportation seemingly ‘a fundamental means of communication between neurons and between neurons and non-neuronal cells’ which strongly influences the ‘plasticity of the nervous system’, according to Korr’s research (Korr 1981).
Patterson & Wurster (1997) note that substances known as nerve growth factors (NGFs) are supplied to the neural structures by the target (end) organ to which neurotrophic substances are being transported. ‘If the end organ does not supply the NGF, the synaptic contact is lost.’ They continue: ‘Complete withdrawal of NGF or of the material delivered by the nerve to its end organ may result in loss of function … The occurrence of the tissue tensions and fluid flow disturbances often associated with somatic dysfunctions can be factors in altering axoplasmic flows.’
Butler (1991) reports that there are two speeds of delivery of trophic substances via the nerves:
Korr (1981) demonstrated that red (postural) and white (phasic) muscle fibres, which differ morphologically, functionally, chemically and, as we will discover later in this chapter, in their response to stress, can have all of their characteristic differences reversed if their innervation is ‘crossed’, so that red muscles receive white muscle innervation and vice versa. ‘This means, in effect, that the nerve instructs the muscle what kind of muscle to be, and is an expression of a neurally mediated genetic influence’, according to Korr (1981).
Neural influences on gene expression
Korr’s research (1981) therefore suggests that it is the nervous system that largely determines which genes in a muscle will be suppressed, and which expressed, and this information is carried in the material being transported along the axons. (See Box 1.2 for additional influences on gene expression.)
Box 1.2 Gene expression
Korr (1981) demonstrated that obstruction of axonal transport modifies gene expression. Additional modulation of gene expression is now known to derive from biomechanical influences, specifically the status of minute structures – integrins – that penetrate the cell surface, acting as a communicating mechanism between the extracellular and intracellular environment.
‘Integrin molecules carry tension from the extracellular matrix, across the cell surface to the cytoskeleton which behaves as a tensegrity matrix’ (Wang et al 1993).
‘Of particular interest are the roles of the integrins in the migration of cells that defend the body against disease and repair injuries’ (Horwitz 1997, Hynes 1992).
The precise effects of tense, distorted, contracted, fibrosed or otherwise dysfunctional tissues on the integrins of the cells in these tissues, and subsequent gene expression, are topics for research, as are the potentially beneficial influences of appropriate bodywork designed to normalize such tissues (Oschman 2000).
Moving beyond neural and mechanical influences on gene expression, Martin (2001) interviewed functional medicine expert Jeffrey Bland, who observed:
Korr (1981) also describes just how vulnerable these highways of nutrition are:
What could cause such neurotrophic interference?
Maitland and Butler: ‘abnormal neural movement’
Butler & Gifford (1989), building on the original work of Maitland (1986), have shown how what they term ‘adverse tension’ in the nervous system can impair its mobility and elasticity, and how many painful problems can result from this. Butler and Gifford’s detailed analysis of the diagnosis and treatment of such restrictions and tensions is highly recommended to manual therapists. The tissues that surround neural structures are known as the mechanical interface (MI). These adjacent tissues are those that can move independently of the nervous system; for example, supinator muscle is the MI to the radial nerve, as it passes through the radial tunnel.
There is no general agreement as to the terminology that should be used in describing such biomechanical changes in the neural environment. Maitland et al (2001), for example, suggest that ‘abnormal neural movement’ is a more accurate description than ‘neural tension’.
Any pathology in the MI can produce abnormalities in nerve movement, resulting in tension on the neural structure with unpredictable ramifications. Examples of MI pathology include nerve impingement by disc protrusion, or osteophyte contact, and carpal tunnel constriction. These problems would be regarded as mechanical in origin as far as the nerve restriction is concerned. Any symptoms resulting from mechanical impingement on neural structures will be more readily provoked in tests that involve movement rather than pure (passive) tension (Alshami & Hodges 2006).
Pathophysiological changes resulting from inflammation, or from chemical damage (i.e. toxicity), are noted by Butler & Gifford (1989) as commonly leading on to internal mechanical restrictions of neural structures in a different manner to mechanical causes such as those, for example, imposed by a disc lesion.
Korr (1970) states:
From the perspective of the manual therapist, this knowledge is extremely important.
The role of neuromuscular techniques in management of adaptive overload
This book has, as a primary focus, the use of neuromuscular techniques (NMTs) in assessing and treating somatic dysfunction. The objectives of neuromuscular technique (NMT as practised in Europe) and neuromuscular therapy (NMT as practised in the USA) are summarized in Box 1.3.
Box 1.3 NMT: European (Lief’s) neuromuscular technique and American neuromuscular therapy (Chaitow & Delaney 2000)
Neuromuscular technique, as the term is used in this book, refers to the manual application of specialized (usually) digital pressure and strokes, most commonly applied by finger or thumb contact. These digital contacts can have either a diagnostic (assessment) or therapeutic objective, and the degree of pressure employed varies considerably between these two modes of application. There are subtle differences between the European and American versions of NMT. Detailed descriptions of Lief’s NMT will be found in Chapter 6, while the American NMT version is outlined by Judith DeLany in Chapter 10.
Aims of NMT
• deactivate myofascial trigger points
• prepare for other therapeutic methods such as exercise or manipulation
• relax and normalize tense, fibrotic muscular tissue
• enhance lymphatic and general circulation and drainage
• simultaneously offer the practitioner diagnostic information.
NMT attempts to address a number of features that are all commonly involved in causing or intensifying pain and dysfunction (Chaitow & DeLany 2000) including, among others:
• biochemical features – nutritional imbalances and deficiencies, toxicity (exogenous and endogenous), endocrine imbalances (e.g. thyroid deficiency), ischaemia, inflammation
• pyschosocial factors – stress, anxiety, depression, etc.
• biomechanical factors – posture, including patterns of use, hyperventilation tendencies, as well as locally dysfunctional states such as hypertonia, trigger points, neural compression or entrapment.
NMT sees its role as attempting to normalize or modulate whichever of these (or additional) influences on musculoskeletal pain and dysfunction can be identified in order to remove or modify as many aetiological and perpetuating influences as possible (Simons et al 1999), without creating further distress or requirement for excessive adaptation.
General adaptation syndrome (GAS) and local adaptation syndrome (LAS), and connective tissue
Selye (1976) called stress the non-specific element in disease production. In describing the relationship between the general adaptation syndrome (GAS) – i.e. alarm reaction, resistance (adaptation) phase followed by the exhaustion phase (when adaptation finally fails), which affects the organism as a whole – and the local adaptation syndrome (LAS), which affects a specific stressed area of the body, Selye also emphasized the importance of connective tissue. He demonstrated that stress results in a pattern of adaptation, individual to each organism. He further showed that, when an individual is acutely alarmed, stressed or aroused, homeostatic (self-normalizing) mechanisms are activated – this is the alarm reaction of Selye’s general (and local) adaptation syndromes.
If the alarm status is prolonged or repetitive, defensive adaptation processes commence and produce long-term – chronic – changes. In assessing (palpating) the patient, these neuromusculoskeletal changes represent a record of the attempts on the part of the body to adapt and adjust to the stresses imposed upon it as time passes. The results of repeated postural and traumatic insults of a lifetime, combined with changes of emotional and psychological origin, will often present a confusing pattern of tense, contracted, bunched, fatigued and ultimately fibrous tissue (Chaitow 1989).
The minutiae of the process are not, for the moment, at issue. What is important is the realization that, due to prolonged stress of a postural, psychic or mechanical type, discrete areas of the body become so altered by the efforts to compensate and adapt that structural and, eventually, pathological changes become apparent. Researchers have shown that the type of stress involved can be entirely physical in nature (Wall & Melzack 1991) (e.g. a single injury or repetitive postural strain) or purely psychic in nature (Latey 1983) (e.g. chronically repressed anger). An example of localized emotional stress influences on muscle tissue is given in Box 1.4. Wider biomechanical responses to emotional stress are discussed later in this chapter.
Box 1.4 Selective motor unit involvement
The effect of psychological influences on muscles seems to be more complex than a simplistic ‘whole’ muscle or regional involvement. It has been demonstrated that a small number of motor units in particular muscles may display almost constant, or repeated, activity when influenced psychogenically (Waersted et al 1993). The reaction time taken to perform tasks was evaluated in normal individuals, so creating a ‘time pressure’ anxiety. Researchers were able to demonstrate low-amplitude levels of activity (using surface EMG in trapezius muscles) even when the muscle was not being employed. It seems that, in spite of low total activity level of the muscle, a small pool of low-threshold motor units may be under considerable load for prolonged periods of time.
Such a recruitment pattern would be in agreement with the ‘size principle’ first proposed by Henneman (1957), which states that motor units are recruited according to their size. Motor units with type I (postural) fibres are predominant among the small, low-threshold units. If tension-provoking factors (e.g. anxiety) are frequently present, and the subject repeatedly recruits the same motor units, overload may follow, possibly resulting in a metabolic crisis and the appearance of type I fibres with an abnormally large diameter, or ‘ragged-red’ fibres, which are interpreted as a sign of mitochondrial overload (Edwards 1988, Larsson et al 1990). The implications of this information are profound, because they suggest that emotional stress can selectively involve postural muscle fibres that have a tendency to shorten over time when stressed (Janda 1983).
The possible ‘metabolic crisis’ suggested by this research has strong parallels with the evolution of myofascial trigger points as suggested by Simons, a topic that is discussed in greater detail in later chapters (Wolfe & Simons 1992).
As described in this and later chapters, predictable chain reactions of compensating changes will evolve in the soft tissues in most instances of chronic adaptation to biomechanical and psychogenic stress (Lewit 1992). Such adaptation is almost always at the expense of optimal function, as well as being an ongoing source of further physiological embarrassment.
A biomechanical stress response sequence
(Basmajian 1974, Dvorak & Dvorak 1984, Janda 1982, 1983, Korr 1978, Lewit 1999, Travell & Simons 1983, 1992, Liebenson 2006, Key 2007, Vleeming et al 2007)
• ‘Something’ (see Causes of soft tissue dysfunction below) occurs that leads to increased muscular tone.
• Increased tone, if anything but short-term, leads to a retention of metabolic wastes.
• Increased tone simultaneously leads to a degree of localized oxygen lack (relative to the efforts being demanded of the tissues) – encouraging ischaemia.
• Increased tone might also lead to a degree of oedema.
• These factors (retention of wastes, ischaemia, oedema) result in discomfort or pain.
• Discomfort or pain leads to increased or maintained hypertonicity.
• Inflammation, or at least chronic irritation, may be a result.
• Neurological reporting stations in hypertonic tissues will feed the CNS with information regarding their status, leading to a degree of sensitization of neural structures, and the evolution of facilitation – hyperreactivity (see Ch. 2).
• Macrophages are activated, as is increased vascularity and fibroblastic activity.
• Connective tissue production increases, with cross-linkage, leading to shortened fascia.
• As all fascia/connective tissue is continuous throughout the body, any distortions that develop in one region can potentially create distortions elsewhere, so having a negative influence on structures that are supported by, or attached to, the fascia, including nerves, muscles, lymph structures and blood vessels.
• Changes occur in the elastic (muscle) tissues, leading to chronic hypertonicity and, ultimately, to fibrotic changes.
• Hypertonicity in a muscle will produce inhibition of its antagonist muscles.
• Chain reactions evolve in which some muscles (postural – type I) shorten, while others (phasic – type II) lengthen.
• Because of sustained increased muscle tension, ischaemia in tendinous structures occurs, as it does in localized areas of muscles, and periosteal pain areas develop.
• Abnormal biomechanical effects occur, involving malcoordination of movement – with antagonist muscle groups becoming hypertonic (e.g. erector spinae) or inhibited (e.g. the rectus abdominis group).
• The firing sequences of antagonistic and synergistic muscles alter.
• Joint restrictions and/or imbalances as well as fascial shortenings develop.
• Progressive evolution of localized areas of hyperreactivity of neural structures occurs (facilitated areas) in paraspinal regions or within muscles (trigger points).
• The degree of energy wastage due to unnecessarily maintained hypertonicity leads to generalized fatigue.
• More widespread functional changes develop – for example affecting respiratory function – with repercussions on the total economy of the body.
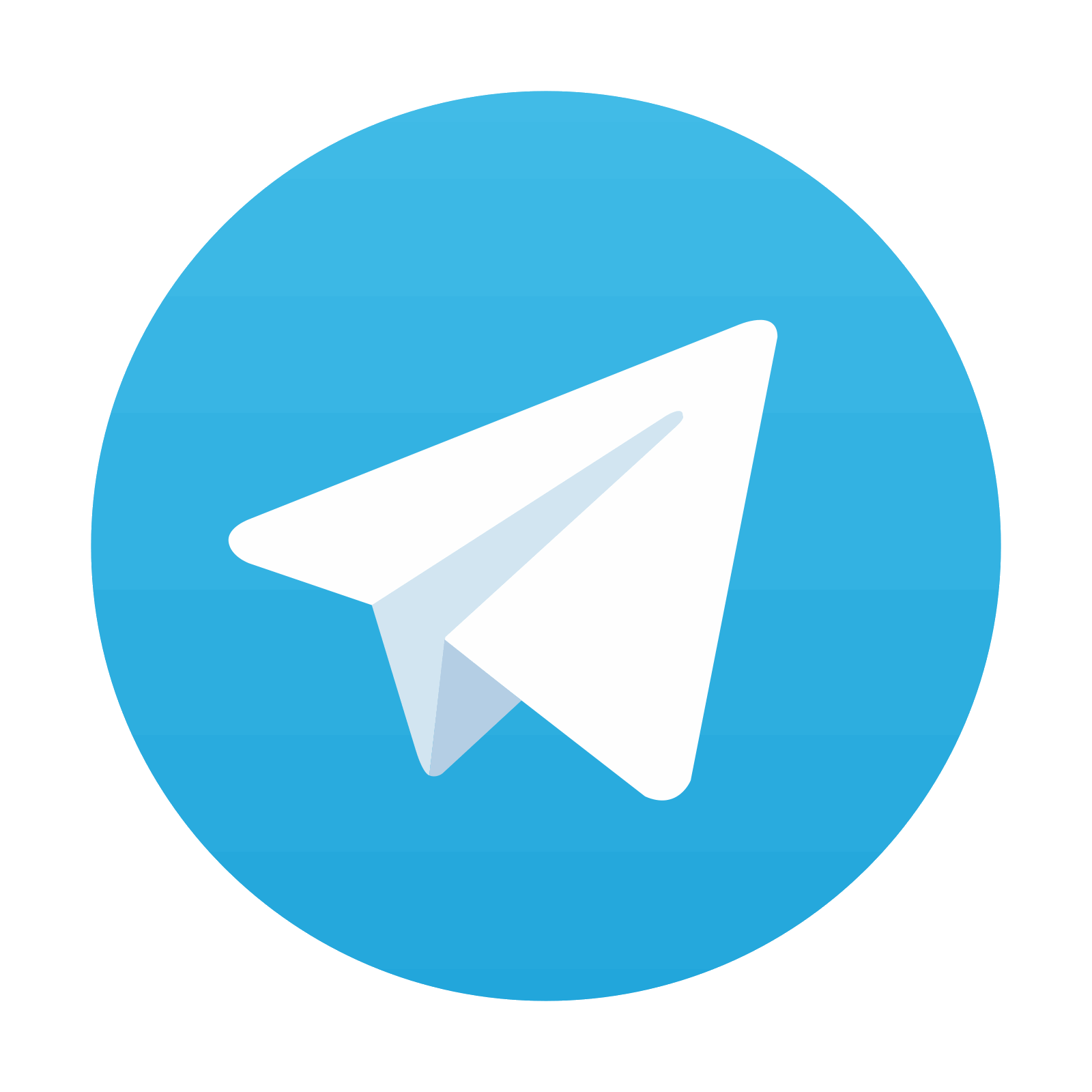
Stay updated, free articles. Join our Telegram channel
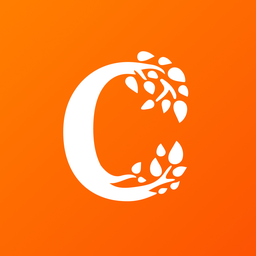
Full access? Get Clinical Tree
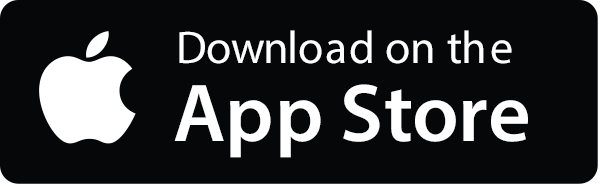
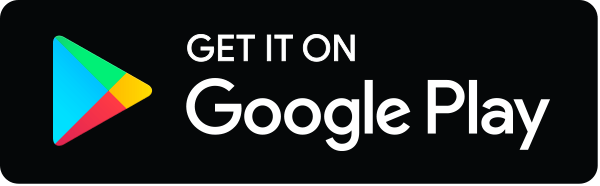