Fig. 8.1
High resolution CT image of a diploic human skull showing inner table, outer table and diploe
8.2 Anatomy
From a biomechanical perspective, the human skull bone lies inferior to the scalp, a connective tissue [9]. It is generally composed of three layers: the outer and inner tables sandwiching a diploe layer (Fig. 8.1). Structurally, the diploe is soft, with material properties similar to the cancellous bone of the human vertebral column, and the two tables are relatively rigid, comparable to the cortical bone of the vertebrae or long bones [10]. The bones of the calvarium, the frontal, occipital, parietal, temporal, sphenoid and ethmoid (not shown) bones are regionally connected by interdigitated sutures, knit since childhood with adult structural properties similar to the nearby diploic skull (Fig. 8.2). The geometry of the cranial vault is complex and three-dimensional. Generally, the cranial vault is symmetric about the mid-sagittal plane. The temporal region is concave medially and the parietal region is convex. This changing geometry including thickness variations, often challenges, investigators to accurately, load or impact one region without engaging the other. Despite geometrical differences, skull bones are constitutionally similar. Dura separates the inner table from the brain.
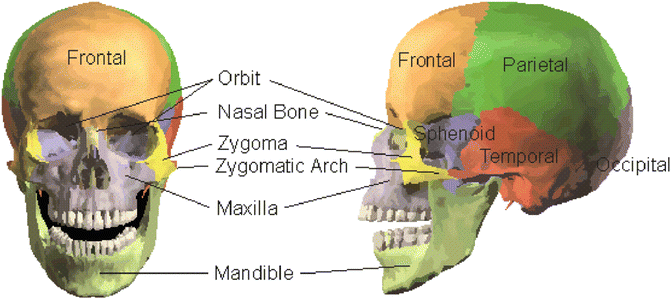
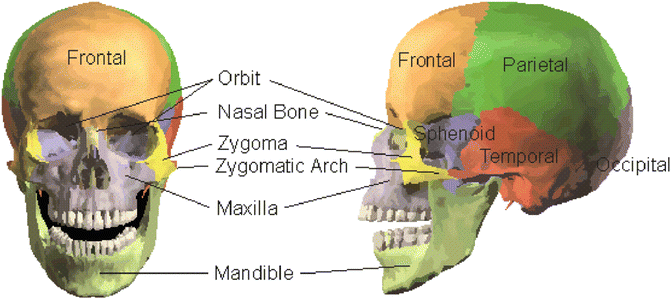
Fig. 8.2
Bones of the skull on the left and facial bones including the orbit on the right
The upper facial skeleton (Fig. 8.2) is comprised of a complex system of interconnected bones that articulate with the skull [11, 12]. The principal bones studied include the maxilla, zygomatic bone, nasal bone and orbit. The maxilla is in the mid-face and supports both the orbital floor and the upper mandible. Dentition of the upper mandible resides in the alveolar process. The zygomatic bone, or cheek bone, articulates with the maxilla and forms the prominence of the cheeks, known as the malar eminence. The zygomatic arch extends posteriorly where it articulates with the temporal bone. The frontal bone forms the forehead, and it is connected with the nasal bones, maxilla, and the zygomatic bone. The orbit is a generally conical cavity comprised of several bony structures. The frontal bone extends to the superior wall of the orbit while the maxilla extends to the orbital floor. The zygoma extends to the orbit lateral wall. The lacrimal, ethmoid and sphenoid bones form the remainder of the orbit. The medial and inferior walls are extremely thin. Fractures of the bones of the orbit walls are usually produced from two sources or combinations of these two. The first is fractures of the maxilla, zygoma or frontal bone that continue into the orbit. The second is, indirect contact from the globe of the eye, also known as blowout fractures. These fractures may result in subsequent herniation of the globe.
The mandible (or lower jaw) is biomechanically complex [13]. The seat of the lower dentition, the mandible is mechanically articulated (hinged) at the condyle with muscular insertions at the coronoid process (Fig. 8.3). Though the mandible is the strongest bone in the face, the articular dislocation force is not large, especially in the absence of muscular control [14–16]. The major functions of the mandible in humans are eating and modulation of speech.The maximum bite force is generally large, 200–400 N in adult males [17, 18] and approximately 100–200 N in adult females [18].
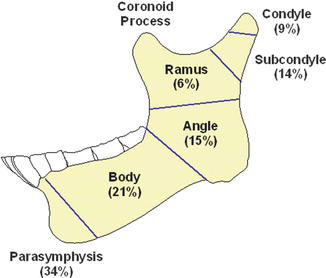
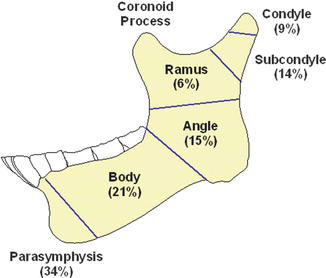
Fig. 8.3
Anatomy of the mandible and typical fracture locations
8.3 Injury Classification/Severity Scales
Several injury severity scales have been devised for grading skull/facial fractures. These include the all-encompassing Injury Severity Score based on the AIS (Abbreviated Injury Scale, AIS), a threat to life scale with limited granularity for facial fractures [19, 20]. The LeFort Classification grades maxilla fractures into three categories based on the extent of the fracture [21]. LeFort I is a horizontal fracture of the alveolar process. LeFort II is fracture where the body of the maxilla is separated a from the facial skeleton and is pyramidal in shape, and LeFort III is a complete separation of the maxilla from the skull. This system, developed for military blunt trauma, is appropriate for large area fracture but is generally inadequate for localized injuries as it was developed in the early 1900s for military applications – typically blunt trauma to the face over a wide area.
Zingg et al. developed an injury classification based on facial fracture patterns and locations. Incomplete fractures are characterized as Type A, fracture of the zygomatic arch is classified as A1, lateral orbital wall fracture is A2 and infraorbital rim fracture is A3 [22]. Complete monofragment fractures are Type B in which the zygoma is completely separated at all three sites. Multifragment fractures are Type C in which the zygoma is separated and the body is comminuted. This classification scheme does not generally address the severity of fracture patterns. Donat et al. defined a system that hypothesizes six notional horizontal beams and five notional vertical buttresses supporting the facial bones [23]. Fractures are described using disruption of support segments. This method does not well represent the structural support of the facial bones and is not strictly a severity rating, but is useful as a descriptive tool for physicians. Rhee et al. developed a classification similar to the AIS for facial bones, ranging in severity from 1 (no fracture) to 6 (displaced zygomaticomaxillary fracture with significant concomitant fractures) based on subjective measures [24]. Unlike the AIS, it is applicable to facial fracture alone, allowing finer distinctions between similar injuries.
There are a number of isolated mandible fracture severity classifications. Joos et al. developed a mandibular fracture score ranging from 0 to 15 based on preoperative factors (location, displacement, fracture complexity and systemic factors) and intraoperative factors, (difficulty of reduction, undefined occlusion and difficulty of soft tissue coverage [25]. This score was found to be well correlated with complication rates (binary classification) with R2 value of 0.88. Zhang et al. proposed the Maxillofacial Injury Severity Score (MFISS) based on AIS as the product of the three highest AIS scores in the maxillofacial region combined with three functional parameters [26]. This score showed significant correlation between the MFISS score and days of hospitalization, but the correlation coefficients were quite low (e.g., 0.26 for cost). More recently, Shetty et al. developed the UCLA Mandible Injury Severity Score [27]. This scale is based on a multiple level scale of fracture characterizations. Constituent scaled fracture variables in this composite score include fracture (F) type, fracture location (L), occlusion (O), soft tissue involvement (S), presence of infection (I) and interfragmentary displacement (D). Ranging in value between 0 and 21, MISS shows a statistically significant correlation with sensory nerve deficits, hospitalization and pain, but no significant correlation with postoperative complications.
8.4 Biomechanical Studies
8.4.1 Skull (Cranial Vault)
Gurdjian et al. dropped intact heads from cadavers onto a solid steel slab [28]. Strain sensitive lacquer was used to identify the pathology [29]. Linear fractures occurred due to tensile stresses from local skull bending. Energies ranged from 650 to 1,230 Nm. Velocities ranged from 4.6 to 6.3 m/s. Fracture limits for dry skulls were lower than for intact heads and comparison of data with static tests demonstrated loading rate effects [30]. To study the effects of contact area on bone tolerance, Nahum et al. delivered impacts using an impacting mass to the temporo-parietal junction of ten PMHS heads [31]. Average fracture forces were lower in females (3,123 N ± 623) in males (3,944 N ± 1,287). Minimum thresholds of 2,450 N for males and 2,000 N for females were suggested for clinically significant fractures. In a follow up study, Schneider et al. conducted additional temporo-parietal impact studies using six intact and nine isolated head-C7 PMHS [32]. Intact PMHS were supine and isolated specimens were supported by wedges of polyurethane padding. Weights were dropped at velocities from 3 to 6 m/s. Injuries ranged from none to severe comminuted fractures. Comparison of the temporo-parietal data with frontal bone tolerance (Fig. 8.4) from tests conducted showed that peak forces for the two regions fall within the range of each other.
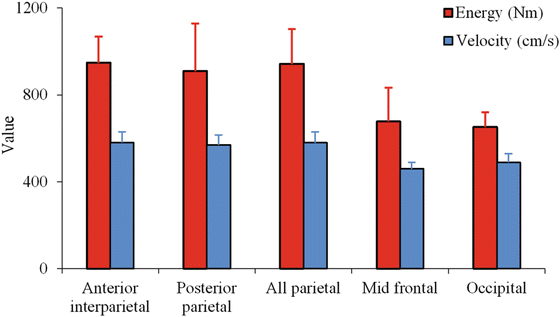
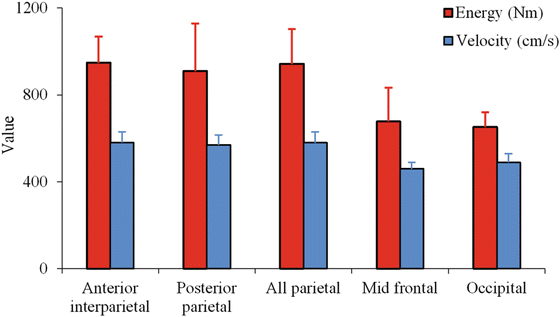
Fig. 8.4
Energy and velocity parameters for skull fracture (Data from Gurdijian et al. 1943)
Hodgson and co-workers investigated the biomechanics of frontal, lateral, occipital and facial regions of embalmed PMHS [33–35]. Results were reported from 35 drop tests conducted with seven male PMHS [34]. Two pairs of bi-axial accelerometers were corded to record transverse and antero-posterior (AP) skull accelerations. A load cell mounted under the rigid impact surface recorded the forces. The head was restrained by a cord from rotating into the preferred position during free fall. The PMHS were raised to the desired height and dropped (1.6 to 4.7 m/s) to contact a rigid flat plate. One specimen was dropped on the left side four times and on the right side nine times without fracture. All other six specimens showed linear fractures. Peak impact forces ranged from 5,560 to 17,792 N (mean: 10,151 ± 4,928). Peak AP accelerations ranged from 190 to 325 g (mean: 267 ± 71). Pulse times ranged from 2.5 to 6 ms (mean: 4.6 ± 1.5). These values were lower than those from frontal impact tests. A comparison of side impact force (Fig. 8.5) and acceleration (Fig. 8.6) data with their companion study on rear and frontal bones indicated the skull to be the strongest in the posterior, followed by the lateral and frontal regions [36].
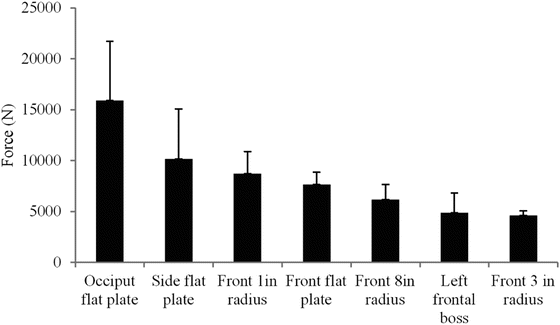
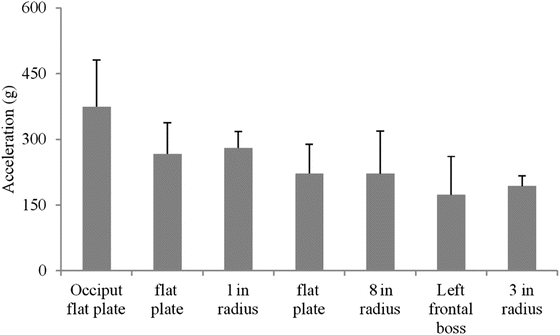
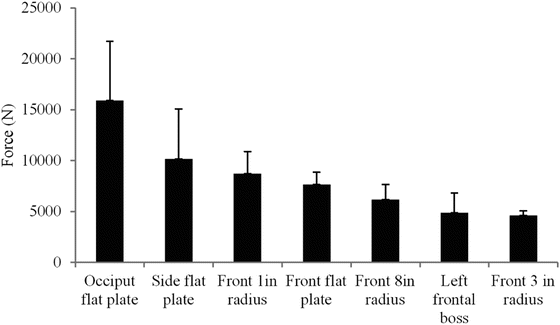
Fig. 8.5
A comparison of peak side impact forces with data from rear and frontal bones (Data from Hodgson et al. 1972)
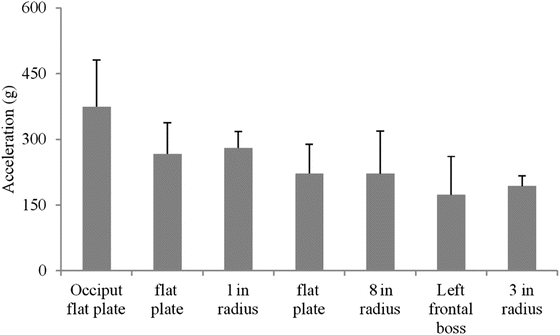
Fig. 8.6
A comparison of peak side impact acceleration with data from rear and frontal bones (Data from Hodgson et al. 1971)
McElhaney et al. reported data from sub-failure quasi-static tests conducted using intact PMHS heads [37]. The head was positioned between two steel platens. Force–deflection curves from loading to the temporal and frontal regions were reported from 12 tests. From the non-linear force–deflection responses, bilinear approximations were made, and the stiffness in the second region ranged from 700 to 1,750 N/mm for the temporal and 1,400 to 3,500 N/mm for the frontal regions. Significant overlap existed (Fig. 8.7) in the response between the two anatomical sites. Further, both sites responded with forces ranging from 4,000 to 8,000 N. Viano et al. investigated frontal bone fracture at high velocities to 69 m/s finding peak forces ranging from 2,450 to 4,080 N without producing fracture [38]. This is consistent with the results of McElhaney, but it is unknown whether fracture force tolerances for the frontal bone are higher at higher velocities.
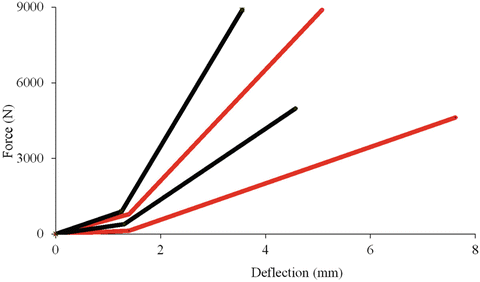
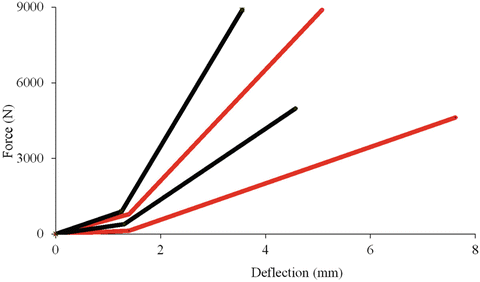
Fig. 8.7
Force–deflection responses for the side (red) and frontal (black) regions of the human head showing the range of responses bounded by the two respective curves (Redrawn from Ref. [37])
Stalnaker et al. conducted left lateral impacts on PMHS seated in the upright position using a pneumatic piston [39]. Three padded and two rigid impacts were conducted on one male and four female PMHS. Peak forces and accelerations ranged from 4.21 to 9.59 kN (mean: 6.1 ± 2.3) and 125 to 532 g (mean: 247 ± 169). All three drops with padded surfaces did not result in bony pathology with peak forces ranging from 4.2 to 4.8 kN and peak accelerations from 125 to 179 g. However, both rigid drop specimens sustained AIS 3 fractures: temporal and occipital bones in one case at a peak force of 7.15 kN and acceleration of 262 g, and a comminuted fracture of the temporal bone at a peak force and acceleration of 9.6 kN and 532 g in another case. Impact velocities were 7.2 and 6.8 m/s for these two impacts. Padded impacts resulted in lower forces and accelerations than did rigid impacts, with lower pulse durations. Impact tolerance to the side head was found to be approximately 5 kN.
The temporo-parietal responses were determined from five intact PMHS subjected to 19 rigid and padded impacts [40]. Three drop heights were used: 1.83 m according to the United States Federal Motor Vehicle Safety Standards, FMVSS 218, 2.5 m according to the French standard and 3 m. Two rigid impact tests produced peak forces of 12.2 and 12.5 kN, and three tests with padded impact produced peak forces ranging from 5.0 to 10.1 kN. Although no fractures were identified in padded impacts, both specimens in rigid impacts sustained skull fractures. In one rigid impact, HIC exceeded 7,000 with frontal and exceeded 1,700 with right temporal accelerometer data. The use of the contralateral accelerometer produced minimal variations in HIC while the use of computed center of gravity (CG) accelerations increased the HIC by a factor of 2.5; the force increased by approximately 25 %. The HIC computed from the accelerometer placed on the right side did not exhibit the same tendency (high or low) when compared with the HIC from sensors placed at other anatomic locations or computed at the CG. However, HIC computed from the CG accelerations were always lower than those computed from the frontal accelerometer.
Impact tests using the frontal bone as the site of loading (7.7 m/s) produced no fractures in six specimens with peak forces from 5.6 to 14.0 kN. HIC computed using the CG acceleration data were 1,000 and 2,800 for two specimens (forces 5.6 and 6.0 kN). HIC computed using rear accelerometer signals ranged from 1,022 to 3,870. The force data matched very well with such data for the side loading suggesting the overlap phenomenon between the fracture thresholds for these sites. However, such clear conclusions could not be drawn for fracture tests because of differences in impact velocities. In a later study, these investigators reported poor correlations (R < 0.2) between age, cranial vault dimensions and fracture force [41]. Higher correlations were found between vault weight and mineralization (R = 0.74). Skull bone condition factor, defined using the thickness of the skull, skull diameter, skullcap mineralization and head mass, correlated well with mechanical tests performed on skullcap fragments.
Nahum et al. conducted tests using one embalmed (four impacts) and five unembalmed PMHS (one impact to each cadaver) using a rigid mass at velocities from 6.5 to 7.5 m/s for the embalmed cadaver and from 7.0 to 10.2 m/s for unembalmed specimens [42]. Peak head accelerations correlated well with HIC (334–1,466 for embalmed and 1,340–5,246 for unembalmed cadaver tests). The time interval maximizing HIC was considerably lower for unembalmed than for embalmed specimens. Peak head accelerations linearly correlated (R2 = 0.86–0.99) with positive pressures in the right frontal area near the region of impact (R2 = 0.99).
Allsop et al. conducted impact tests on 31 isolated unembalmed PMHS heads [43]. The specimens were impacted using flat rectangular (at 4.3 m/s) or circular (at 2.7 m/s) plates. The impactors were fixed to a drop tower. Two locations on the temporo-parietal regions were selected for the circular impactor. For the rectangular impactor, the parietal region was selected as the impact site. The mean fracture for rectangular plate impacts was 12,390 N (±3,654). The average fracture force for both impact sites with the circular impactor was 5195 N (±1010). Rectangular plate tests responded with stiffness ranging from 1,600 to 6,430 N/mm (mean: 4,168 ± 1,626). Circular plate tests resulted in stiffness values ranging from 700 to 4,760 N/mm (mean: 1,800 ± 881). The contact area of the impactor significantly affected peak forces. Hodgson et al. and Yoganandan et al. advanced similar conclusions for facial bone structures in experimental studies in the 1970s and 1980s [11, 12, 34, 44, 45].
McIntosh et al. conducted lateral impacts to 11 unembalmed PMHS using an aluminum impactor [46]. Tests at velocities from 3.9 to 6.1 m/s were conducted without padding; tests at velocities from 2.8 to 3.8 m/s were conducted with padding in front of the impactor. Skull fractures occurred in three undamped impacts at >4.0 m/s and AIS ranged from 2 to 4. Peak biomechanical parameters were lower in specimens with no injury (AIS = 0) compared to injured specimens. Head mass was scaled according to procedures outlined by Reynolds [47]. Because of the limited sample size, data from occipital impacts (four fracture and four non fracture) was grouped with side impact data, and risk curves were developed using a logistic regression analysis. At the 200 Hz filter level, a HIC value of 800 represented 50 % injury risk.
Yoganandan et al. conducted studies by fixing the inferior end of the intact PMHS head using a custom-designed device and applying static and dynamic loads with the piston of the electro-hydraulic testing apparatus [48]. Failure forces were 5,292 and 5,915 for the two parietal loading specimens and 6,182 N for the temporally loaded specimen. Temporal and parietal fractures were reported. Failure deflections for these specimens were 8.9, 7.8 and 15.4 mm, and stiffness was 695, 1,143 and 487 N/mm. The parietal region was stronger than the temporal region, although the temporal bone was more compliant. Force tolerance increased by approximately a factor of two under dynamic loading, while the stiffness increase was higher (Fig. 8.8). While x-rays and computed tomography scans identified skull fractures, the precise location and direction of the impact on the skull were not apparent in these images. The authors concluded that, based on retrospective imaging, it may not be appropriate to extrapolate the anatomical region that sustained the external insult. Because the experimental design included monitoring forces and deflection, actual force-deflection plots were provided.
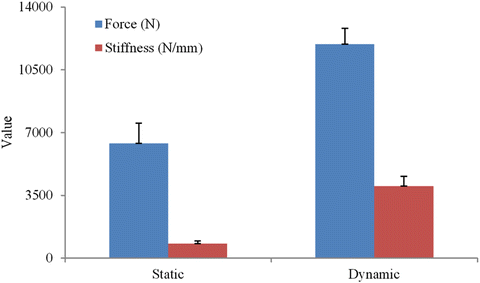
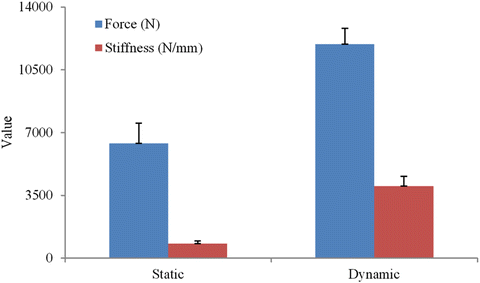
Fig. 8.8
Comparison of force and stiffness at two different rates of loading from isolated specimens (Adapted from Ref. [48])
McElhaney et al. conducted a study to investigate three mechanisms of basilar skull fractures using human cadaver specimens [49]. These include transmission of loading from the mandible through the temporomandibular joint (TMJ) in a anteroposterior (AP) direction, direct TMJ loading with neck tension simulating inertial loading and direct superior-inferior cranial vault impact [50]. They found no evidence that direct transmission in the AP direction through the TMJ results in basilar skull fracture, but did produce basilar skull fracture with applied neck tension with loading vertically through the TMJ with force levels ranging from 2,590 to 4,880 N. Of the 11 tests with loading applied vertically by impacts to the cranial vault, 7 tests produced cervical spinal fractures while only one produced a basilar skull fracture in concert with a cervical spinal fracture.
8.4.2 Facial Bones
Many of the investigations of facial fracture are associated with automobile safety, aimed at assessing injury from contact with the steering column, dashboard, windshield or air bag. For example, a Society for Automotive Engineers (SAE) task force summarized available data to 1986 for facial fracture forces [51]. The focus of these and more recent investigations has been generally direct impact on the maxilla, zygoma and blowout fractures of the orbit.
Schneider et al. conducted 13 maxillary impacts and 27 zygomatic impacts on 17 human cadavers using a circular disc impactor with a surface area of 6.5 cm2 with various velocities and masses [32]. Eleven of the maxilla impacts produced fractures. The peak force to fracture ranged from 625 to 1,980 N. Seventeen of the zygoma impacts produced fractures with the peak force ranging from 930 to 2,850 N. A series of 113 impact experiments to various facial bones were performed by Nahum using both male and female cadavers that were both embalmed and unembalmed [52, 53]. Maxilla and zygoma were found to have approximately 25 % of the impact strength of the frontal bone for a 6.5 cm impactor (Table 8.1). Karlan and Cassisi studied fractures of the body of the zygoma, treating the structure as a flattened pyramid with multipoint support [54]. Stanley and Nowak emphasized the importance of impacts to the buttressing structures of the zygoma and maxilla in creating more severe facial fracture. Nyquist et al. reported 11 impacts to the nasal bones of human cadavers [55]. They found an association of impact kinetic energy with fracture severity. Impact forces greater than 3 kN were associated with fractures of the maxilla, zygoma and frontal bone. Impact kinetic energy varied from 241 to 815 J.
Table 8.1
Summary of experimental studies of facial fracture
Author (year) | Number of specimens | Impact location | Impactor geometry | Impactor area (cm2) | Initial energy (J) | Velocity (m/s) | Peak force (N) |
---|---|---|---|---|---|---|---|
Schneider (1972) | 13 | Maxilla | Circular | 6.5 | 4.9–26.3 | 3–5.4 | 625–1,980 |
27 | Zygoma | 6.4–46.7 | 3–5.2 | 930–2,850 | |||
Nahum (1975a) | 9 | Maxilla | Circular | 6.5 | 4.9–26.3 | 3–5.4 | 625–1,980 |
16 | Zygoma | Circular | 6.5 | 12.6–86.1 | 2.2–7.5 | 610–3,470 | |
9 | Zygomatic arch | Circular | 6.5 | 6.4–46.7 | 3.0–5.4 | 930–1,940 | |
21 | Frontal bone | Circular | 6.5 | 25.3–133.8 | 3.1–7.1 | 2,670–9,880 | |
8 | Lateral mandible | Flat | 25 (2.5 × 10 cm) | 56.9–68.4 | 5.4–6.0 | 820–3,400 | |
9 | Mandible symphysis (AP) | Circular | 6.5 | 37.3–46.7 | 4.9–5.4 | 1,900–4,100 | |
Nyquist (1986) | 11 | Nasal bone | Cylinder | 5 | 240–815 | 2.8–7.1 | 2,000–4,500 |
Allsop (1988) | 6 | Maxilla | Cylinder | 2 cm diameter bar | 43–87 | 2.4–3.5 | 1,000–1,800 |
8 | Zygoma | 900–2,400 | |||||
Yoganandan (1988) | 9 quasistatic | Zygoma | Steering wheel | 0.0025 | 1,200–1,400 | ||
15 dynamic | 2.0–6.9
![]() Stay updated, free articles. Join our Telegram channel![]() Full access? Get Clinical Tree![]() ![]() ![]() |