, David B. Burr2, Neil A. Sharkey3 and David P. Fyhrie4
(1)
Orthopaedic Surgery, UC Davis Medical Center, Olga, WA, USA
(2)
Department of Anatomy and Cell Biology, Indiana University School of Medicine, Indianapolis, IN, USA
(3)
Kinesiology, Orthopedics and Rehabilitation, Pennsylvania State University, University Park, PA, USA
(4)
Orthopaedic Surgery, Biomedical Engineering, UC Davis Medical Center, Sacramento, CA, USA
Abstract
Chapter 1 has shown us that the skeleton must withstand very high forces; because our muscles can only contract a small percentage of their length, we must amplify movements using levers that “spend rather than save forces,” as Borelli put it. In this chapter we begin to develop an understanding of how the biology of bone, cartilage and the other connective tissues provides tissues that can support these large forces day in and day out for a lifetime.
Keywords
BoneCartilageTendonLigamentStructureCompositionGrowthThe human skeleton is a mechanically optimized biological system whose composition and organization reflect the functional demands made on it.
Thomas Einhorn (1992)
Nothing in biology makes sense except in the light of evolution.
Theodosius Dobzhansky (1973)
2.1 Introduction to Bone
As more is learned about the mechanics of the skeleton, it becomes increasingly clear that mechanical demands heavily influence what happens biologically. Most of what we know about the basic cell- and tissue-level mechanisms that enable bone and its adjoining connective tissues to adjust to mechanical forces has been learned since the 1960s. Before then, skeletal research was focused on bone chemistry. Calcium is a metal and phosphorus a non-metal that, along with oxygen and hydrogen, are primary constituents of bone mineral. The serum concentrations of calcium and phosphorus are of great significance in physiology and medicine, and there was intense interest in the middle of the twentieth century in how bone contributed to the homeostatic regulation of these elements. Physiologists and endocrinologists realized that bone mineral served as a calcium and phosphorus reservoir for the rest of the body. Bone biology was seen largely in terms of its role in transferring mineral to and from the skeleton. Moreover, it was recognized that bone’s mechanical properties—its strength and stiffness—were to a significant degree determined by the bone’s mineral content. Early work by John D. Currey of the University of York and others demonstrated the strong correlations between bone density and strength (Currey 1969a, 1969b) and, since bone mineral content could be measured in living animals using x-ray methods, it appeared that the main factors that cause bone tissue to be strong or weak were well understood. The roles of collagen alone and of the mineral-collagen composite were investigated to a much lesser degree, partially because collagen content and conformation could not be non-invasively measured in living animals.
Exploring the micro-anatomy of bone and the cellular constituents responsible for maintaining its strength was also challenging. Preparation of undecalcified bone sections entailed the tedious process of cutting thick sections of cortical bone and grinding them by hand using grinding powder or sandpaper to a thickness of 100–150 μm, where accurate analysis could take place. Although this was an employment possibility for orthopaedic residents and graduate students, it severely curtailed the number of specimens that could be made. With the advent of plastic embedding in the 1970s it became possible to cut thin sections using a microtome or diamond saw, greatly improving and accelerating the technical preparation of microscope slides. This fueled research by Ascenzi, Bonucci and others to study the microscopic structure of bone, and relate this to its mechanical properties (Ascenzi and Bonucci 1964, 1967, 1968, 1972).
Bone is much more, however, than its mineralized components. It is a hierarchically-organized material that is constructed as a fiber-reinforced composite material (Fig. 2.1).


Figure 2.1
Bone is arranged hierarchically according to structure at size scales ranging from nanometers (collagen and mineral) to microns (trabecular plates) to milli- and centimeters (the bone as an organ). Structure at each level imparts a unique mechanical adaptation, and the combination across length scales forms a structure that is capable of lasting decades without failing.
Fiber-reinforced composites are often highly anisotropic (having different mechanical properties in different orientations) and can have low transverse strength because of the directionality of the fibers. Such a design can be used to increase strength in the predominant load direction without severely compromising properties in the perpendicular directions. For example, in a long bone like the femur the strong direction is along the femoral shaft and the weaker, but functionally adequate, directions are circumferential and radial. This is a good design for a femur, where most of the loading is along the long axis of the bone. For cancellous bone, the strong direction of the material is parallel to the surfaces of the trabeculae which, once again, makes sense as that is the predominant loading direction.
At the histological level, bone is either dense compact (cortical) bone, or more porous trabecular (cancellous) bone. The tissue that forms these microscopic architectures can be lamellar (sheet-like) or completely disorganized and nearly devoid of identifiable structure. Bone microstructure is often in layers, alternating between regions with highly oriented mineral (lamellae) and adjacent interlamellar regions. The regions with oriented crystals are 20–50 % stiffer than the interlamellar regions, at least in longitudinal compression (Donnelly et al. 2006; Hengsberger et al. 2002; Xu et al. 2003).
At the microscopic level, secondary osteons (Haversian systems) about 200 μm in diameter can act as strong fibers embedded in a matrix composed of interstitial lamellae. Osteons are separated from the matrix by an interface called the cement line that has relatively low shear strength. The functional importance of cement lines lies in their ability to control fatigue and fracture processes, to absorb energy by stopping crack propagation, and to provide viscous damping in compact bone (Burr et al. 1988).
At the nanoscale, bone matrix is composed of cross-linked collagen fibers interspersed with mineral platelets, with noncollagenous proteins that control the assembly and size of these components. This organizational structure produces a composite material with mechanical properties superior to those of any of its constituents, including a remarkable ability to resist structural failure through the fatigue processes incurred by a lifetime of repetitive loading. As a first approximation bone’s elastic properties are attributed to the mineral phase of the tissue and its inelastic properties to the organic (primarily collagen) matrix. This is clearly approximate and does not consider either the interaction of the two phases or the roles that each plays in both the elastic and plastic deformation of bone tissue.
Box 2.1 What Is Hierarchy?
Bone and other connective tissues are made up from patterns of material that people categorize in a descending order of size as: anatomical, histological, molecular and atomic. All of the material patterns at all of the levels of size affect the mechanical properties. When authors write about bone mechanical properties they commonly say that ‘Bone is a hierarchical material.’ The notion that bone or other connective tissues are hierarchical is useful to help explain tissue mechanics since there is a large engineering literature about man-made hierarchical composites. In man-made hierarchical composites, the smaller design features are deliberately included to improve the functions of the larger parts. An example is the small ridges on the reinforcing steel bars used to strengthen the concrete of a bridge: The steel bars are smaller than the bridge and strengthen it and the ridges are smaller than the steel bars and strengthen the coupling between the steel and the concrete. As an inventor one could envision a roughening of the ridges to make them work better. In a hierarchy of size each smaller design feature improves the larger. A grave weakness of the hierarchy analogy for connective tissues is that the concept of design does not apply to a naturally evolved structure—we can’t know whether small features “help” the larger features to perform or if they are there for no reason at all. Evolution (selection) acts on the whole organism rather than on individual features of anatomy. As a result, heritable traits that are benign or even negative (when linked to an important positive trait) can become common in the population even if they don’t have a function. The human tendency to assign purpose and meaning to anatomical features helps in understanding some of the ways that connective tissues function, but our improved understanding does not mean we have identified a deliberate functional design plan (or hierarchy) inherent to the anatomy.
Recently developed techniques have provided the tools needed to study the finer fabric of bone structure. Quantitative image analysis systems have replaced traditional stereology and greatly improved the ability to discriminate small differences in trabecular architecture. Beginning in the 1980s, three-dimensional analysis using micro-computed tomography (μCT) imaging made it possible to investigate full cancellous architecture using both advanced imaging theory and computational stress analyses. With improved spectroscopic imaging it is now possible to evaluate the structure and properties of bone at the molecular level using Fourier Transform Infrared Spectroscopy (FTIR) and Raman spectroscopy. Spectroscopic techniques are used to measure the mass of mineral and many of its characteristics (e.g. crystallinity, carbonate substitutions in the hydroxyapatite lattice) as well as the amount of collagen and its maturity through measurements of the relative content of younger divalent to more mature trivalent collagen cross-links.
Box 2.2 Fourier Transform Infrared Spectroscopy (FTIR) and Raman Spectroscopy
FTIR and Raman Spectroscopy detect molecular vibration to estimate some of the content and properties of the mineral and collagen of bone. FTIR measures infrared light absorption and reemission where Raman spectroscopy measures the energy change of photons that are scattered from the molecules (not absorbed and re-emitted). Both absorption and the Raman scattering depend on the molecular structure and give frequency dependent “peaks” in the raw data that are characteristic of tissue chemistry and structure. In both FTIR and Raman spectroscopy, it is necessary to decompose the signals using some form of Fourier Transform in order to quantify the measured signal.
There are several characteristic peaks associated with connective tissue composition. The Amide I band occurs from 1688 to 1623 cm−1. Amide I results from vibration of a C=O peptide bond which, in connective tissues, occurs primarily in collagen. The Amide I band signals are, therefore, used to measure collagenous content and structure. For example, the ratio of mature (trivalent) to immature (divalent) collagen cross-links can be estimated from the ratio of peak intensities of the Amide I band at 1660 and 1690 cm−1.
In bone or other calcified tissues the properties of the mineral can be evaluated in part by measuring asymmetric stretch of the P-O bond (ν3/ν1, 1200–900 cm−1), and by measuring in-plane bending (ν4 PO4-3, 650–500 cm−1). The ν3/ν1 PO4-3 phosphate peak can become saturated, indicating that a minimal amount of light is reaching the detector at those wavelengths, and some feel that the ν4 PO4-3 band is a more accurate measure of mineral. Crystallinity can be determined as the ratio of the phosphate sub-bands at 1030 cm−1 and 1020 cm−1. The intensities of the bands between wavelengths 905 and 825 cm−1 are determined by mineral crystallinity, structure and ionic substitutions within the apatite crystal. The ν2 carbonate band has been found to be the best for identifying carbonate ions in hydroxyapatite because it is not obstructed by other bands, and provides a sharp peak. Overall mineralization of the tissue is represented as the ratio of phosphate to total protein (ν4 PO4-3/Amide I), whereas carbonate substitutions are measured as the ratio of ν2CO3-2 /Amide I. Both FTIR and Raman have become common and useful tools for measuring changes in bone composition in experimental animals in response to an intervention, treatment, or disease (Carden and Morris 2000; Ruppel et al. 2008; Gourion-Arsiquaud et al. 2009).
The mechanical properties of some of the individual components of bone tissue can now be measured directly. Nanoindentation (Rho et al. 1997) allows one to sample properties at specific locations and interfaces (cement lines, lamellae and the junctions between them) within the bone tissue. Energy dispersive X-ray diffraction techniques such as Small Angle X-ray Scattering (SAXS) and Wide Angle X-Ray Scattering (WAXS) (Gupta et al. 2006; Stock et al. 2011) can be used to measure mineral crystal thickness and spacing between the mineral plates, as well as lattice strains and the elastic modulus of the bone mineral itself. Atomic Force Microscopy (AFM) can now provide high resolution 3D images of surface features and image structure at the level of the collagen fibril (such as collagen spacing) and the mineral platelets (Wallace et al. 2011). In a different mode, AFM can be used to make mechanical measurements of structure at a molecular level.
Evolution has had millions of years over which to find satisfactory solutions to the structural and materials engineering problems of bone. The ultrastructural, microstructural and even macroscopic organization of the constituents of bone interact in ways that reduce stress and allow the structure to sustain itself over a lifetime. Studies of these natural solutions to engineering problems provide insights into design criteria that can improve the way man-made materials function for particular applications (biomimetics).
2.2 The Shapes of Bones
While the shapes of our bones are quite variable, comparisons with those of other animals show that the architecture of a vertebrate skeleton is fairly conserved in nature and stereotypical across species. We usually can recognize a femur or a tibia, and we recognize that they have a characteristic positional relationship, regardless of what animal they came from. Yet within these broad architectural constraints, the skeletal morphology is quite variable, and we can imagine a rubber archetypical skeleton being stretched and molded to create such diverse skeletons as those of a flamingo, a bat, an elephant, a whale, or a human.
Not only is skeletal architecture conserved across species, but so too is the topology of individual bones within a species. Thus, a physical anthropologist can recognize an isolated bone, or even a fragment of a bone, as belonging to a human rather than one of the other primates. These constraints on architecture and topology give the impression that bones are determinate, static structures. On a scale of centimeters, this is approximately true. Yet the specific mechanical behavior of bones also relies on slight variations in the geometric distribution of bone, either within a cross section of compact bone, or by variations in trabecular architecture that correspond to the pattern of locomotion and the directions of the stresses and strains imposed by the animal and its environment. Differences in geometry (gross or macroscopic anatomy) can compensate for differences in the microanatomy (or nanoanatomy) that change the mechanical properties of the tissue. It can be readily appreciated that the strength of a whole bone depends on the material composing it, and its volume, cross-sectional area, three-dimensional shape, ratio of the smallest diameter to total length and other shape characteristics. These gross geometric properties of the bone are complemented by the material properties of the tissues themselves. Tissue material properties also are variable in space and time, responding to mechanical stimuli. The complex interrelationship between the physical properties of bone—those determined by the mineral-collagen composite—and the geometric properties, together determine the overall mechanical behavior of a whole bone as a structure.
2.3 Types of Bone and Tissue
Figure 2.2 is a sketch designed to show the general features found in most long bones, such as the humerus or metatarsal. This figure illustrates the relationships between many of the structural features of bones described in this chapter.




Figure 2.2
(a) Sketch of a hemi-section of bone showing important features three dimensionally of the cortical bone from a typical long bone. (b) Microradiograph (X-ray image of a thin cross section) showing compact bone. Haversian canals and resorption spaces are black; note variable mineralization of osteons. Outermost region (at top) contains well-mineralized primary bone. Field width, ~2 mm (Courtesy of Dr. Jenifer Jowsey).
The nonmineralized spaces within a bone contain marrow, a tissue composed of blood vessels, nerves, and various types of cells. The chief function of the marrow is to generate the principal cells present in blood. The internal presence of marrow is a nearly universal feature of bones (the ossicles of the inner ear are an exception). The relationship of marrow and bone is both biologic and physical: they share common stem cells, and marrow never exists outside of bone. Bone can be made to form outside the normal skeleton (e.g., in a muscle) by implanting osteogenic materials, but when this happens, a space containing marrow automatically forms in the nodule of extraskeletal bone. Moreover, marrow itself is a highly osteogenic material and can stimulate bone formation if placed in an extraskeletal location (Tavassoli and Yoffey 1983).
There are two types of marrow in bone, each with a different function. Bone is a blood forming organ, and red, or hematopoietic marrow, within the marrow cavity is abundant during rapid growth when there is need for an ever increasing volume of red and white blood cells. Red marrow begins to be replaced by yellow (fatty) marrow by the age of 13 as the growth rate slows down, with the red marrow in the diaphyses of long bones being completely replaced by yellow marrow by the middle of the 4th decade of life. The cells in yellow marrow (adipocytes) and bone forming cells (osteoblasts) arise from the same stem cell, creating what can appear to be an inverse relationship between the amount of fat and the amount of bone. In some pathologic conditions the inverse relationship is very strong. Although some red marrow is retained in the ends of the long bones, and in areas characterized by large amounts of cancellous bone (e.g. vertebral bodies, iliac crest and ribs), aging gradually converts more and more of this marrow to yellow or brown marrow.
2.3.1 Cancellous Versus Compact Bones
If you were to slice open a dozen bones of various sorts and wash away the marrow with a high-pressure stream of water, it would be clear that the remaining bone is of two distinct kinds, as determined by porosity (the volume fraction of soft tissues). While in principle the porosity of bone can vary continuously from 0 to 100 %, in fact most bone tissues are of either very low or very high porosity, with little bone of intermediate porosity. These two types of bone tissue are referred to as compact bone and cancellous bone, respectively (Fig. 2.3a).


Figure 2.3
(a) A coronal section of the proximal end of a human femur provides an appreciation for the organization of cancellous bone and its relationship to the dense cortical bone surrounding it. (b) Cancellous bone structure from the distal end of the femur at higher magnification shows more clearly the integration of trabecular rods and plates. Field width, ~1 cm.
Cancellous bone (also called trabecular or spongy bone) is porous bone found inside cuboidal bones (e.g. vertebrae, carpal bones), the flat bones (e.g. ilium, sternum), and the ends of long bones (Fig. 2.3b); its porosity is 75–95 %. The pores are interconnected and filled with marrow. The bone matrix is in the form of plates or rods called trabeculae, each about 100–150 μm thick. The arrangement of the trabeculae is variable. Sometimes they appear to be organized into orthogonal arrays; often, they are more randomly arranged. Cancellous bone tissue has a much lower density than cortical bone and allows the skeleton to build large but lightweight conical volumes of bone at the ends of long bones that carry the large loads of the joints with low stress at the wide end (the metaphysis) and higher stresses in the narrower shaft (the diaphysis). The density of the bone increases as the cone narrows, creating a means for the bone to funnel the high loads imposed at the joint to the stronger and more massive cortical bone comprising the middle portion of the bone (i.e. the diaphysis).
Compact bone is the dense bone found in shafts of long bones and forming a cortex or shell around vertebral bodies and other cuboidal and flat bones (Fig. 2.3a, b). Hence, it is also called cortical bone. It is strong and provides both support and protection. Its porosity is 5–10 %, although this increases with age and with osteoporotic changes to the skeleton. Its pores consist of spaces categorized as follows.
Haversian canals are approximately aligned to the long axis of the bone, contain capillaries and nerve fibers, and are about 50 μm in diameter (about the diameter of a human hair). Haversian canals are named after an English physician, Clopton Havers (1691).
Volkmann’s canals are short, transverse canals connecting Haversian canals to each other and to the outside surfaces of the bone. These canals also contain blood vessels and nerve fibers. They are named after Richard von Volkmann (1830–1889), a surgeon and early advocate of Lister’s antiseptic surgical methods.
Resorption cavities are the temporary spaces created by osteoclasts in the initial stage of remodeling (see Chap. 3). Resorption cavities are about 200 μm in diameter.
It is important to remember that bone is a dynamic porous structure; its porosity may change as the result of a pathologic condition, with age, or in a normal adaptive response to a mechanical or physiologic stimulus. Cancellous bone may become more compact, or compact bone may become more porous. Such changes strongly affect bone’s mechanical properties.
2.3.2 Lamellar Versus Woven Bone
Examining compact and cancellous bone at a still finer scale of resolution, it is evident that each may contain two major types of bone tissue.
Lamellar bone is slowly formed, highly organized bone consisting of parallel layers or lamellae comprising an anisotropic matrix of collagen fibers interdigitated with mineral plates. There may be a variety of patterns of lamellar arrangement at the level of the collagen fibril, and these likely each have important mechanical implications (Reisinger et al. 2011). Microscopically, however, the lamellae create optically bright circumferential bands of bone, each 3–7 μm thick, that give the appearance of tree rings, each separated by an optically darker layer about 1 μm thick that is mechanically less stiff than the thicker lamellae (Tai et al. 2007). The lamellae may be arranged around the endocortical or the periosteal circumference of the bone (primary or circumferential lamellae), or may be arranged concentrically around individual vascular channels (osteonal or concentric lamellae).
Two fundamentally different kinds of “plywood” architecture coexist within the general complexity of bone’s lamellar structure (Giraud-Guille 1988). The first of these schemes corresponds to the classical view of lamellar structure: the collagen fibers are parallel in each lamella and change direction by 90° at the lamellar interface. That is, as a lamella is built up, layers of collagen are put down in one orientation. Then, when the next lamella is started, the orientation of collagen fibers suddenly changes so that they are laid down at right angles to the previous direction. In other regions of the bone, this orthogonal plywood-like structure is replaced by helicoidal plies (Fig. 2.4). In this scheme the collagen fibers continuously change their direction, so that in a sense there are no individual lamellae. However, the bone still shows a lamellar structure because as the orientation of the collagen fibers rotates through 180° cycles, the fiber orientation repeats itself, and layers appear when a histologic section is examined microscopically. A helicoidal arrangement of collagen fibers leads to a transversely isotropic structure at this level of organization as the elastic properties are similar in different directions (Reisinger et al. 2011). In her landmark paper, Giraud-Guille (1988) showed that both these architectures are present in human compact bone, but their relative distribution and interspecies variation have not been determined.


Figure 2.4
Diagram of helicoidal plywood structure. (a) Three-dimensional structure. (b) Visual effect of arches seen when an oblique section (as indicated by the shaded plane in (a) is cut. (c) The arches seen in an oblique section are formed by the helicoidal lamellae (Neville 1984). With kind permission of Springer Science+Business Media.
Both these forms of lamellar structure give rise to birefringence, the capacity of some fibrous structures to interact with polarized light. When a histologic section of bone is transilluminated with polarized light and viewed through a polarizing filter oriented perpendicular to the vibration plane of this incident light, the section appears dark (i.e., the observer sees a “dark field”) except where collagen fibers are parallel to the plane of the section. These collagen fibers rotate the light’s plane of polarization so it is no longer perpendicular to the viewing polarizing filter. Therefore, the light is not blocked and reaches the viewer’s eyes. Thus, in a bone section observed in a polarizing microscope, transversely oriented fibers are bright and longitudinally oriented fibers are dark. In the 1960s, Ascenzi and his co-workers used this phenomenon to categorize osteons as having bright, alternating, or dark lamellae (corresponding to their collagen fibers exhibiting mostly circumferential, alternating, or longitudinal orientation) (Ascenzi and Bonucci 1967) . These classifications are shown as parts a, b, and c, respectively, in Fig. 2.5. A dark “iron cross” pattern superimposed on the brighter osteons shows where the lamellae lie parallel to the polarization directions of the upper or lower polarizing filters.


Figure 2.5
Three osteon types as defined by Ascenzi and Bonucci. Photomicrographs at bottom show appearance in plane-polarized light; diagrams above show hypothesized fiber arrangements in successive lamellae. (a) Type T or transverse (i.e., circumferentially wrapped) fiber orientation; (b) type A or alternating fiber orientations; (c) type L or longitudinal fiber orientation (Ascenzi and Bonucci 1968).
Woven bone is a quickly formed, poorly organized tissue in which collagen fibers and mineral crystals are more or less randomly arranged. Woven bone may become more highly mineralized than lamellar bone, which, mechanically speaking may help compensate for its lack of organization. Woven bone is common in a fracture callus and is formed early in the healing process to help stabilize the broken bone. In this case, woven bone can be considered calcified scar tissue that is rapidly formed for damage control and then remodeled and replaced over time with lamellar tissue reflective of the site’s original organizational structure. The important generalization is that woven bone can be made more quickly than lamellar bone, but is weaker. Rats and other small animals also have fine-fibered bone in the cortices of their bones. In this bone the rather randomly arranged collagen fibers are smaller and closer together than in woven bone, so the tissue appears to be more organized (Fig. 2.6). It may be that the lamellar organization of bone can vary continuously; depending on factors such as how fast it is made.


Figure 2.6
Polarized light image from a rat tibia showing disorganized fine-fibered bone in mid-cortex. This bone appears almost like woven bone, and is composed of very small collagen fibers, randomly arranged. Bone appearing more like primary lamellar bone is found along the periosteal and endocortical surfaces of the bone. Orig. mag. ×10.
2.3.3 Primary and Secondary Bone
Compact bone may be further characterized as primary or secondary bone.
Primary bone is tissue laid down de novo on an existing bone surface, such as the periosteal surface, during growth. It may be of two general types:
Circumferential lamellar bone, in which the lamellae are parallel to the bone surface (Figs. 2.7a–c). Blood vessels are incorporated into the lamellar structure such that each is surrounded by several circular lamellae, forming a primary osteon with a primary Haversian canal at its center.


Figure 2.7
(a) Sketch of primary circumferential lamellar bone structure. Primary osteons form when blood vessels on the bone surface become incorporated into the new periosteal bone. They usually have several concentric lamellae, but their cement line is not scalloped. (b) Photomicrograph of a cross section of a rabbit humerus showing primary lamellar bone running in parallel sheets around the periosteal circumference of the bone. Osteonal bone—demonstrating both primary and secondary osteons can be found adjacent to the circumferential lamellar bone. (c) Bone from a squirrel monkey in polarized light clearly shows primary lamellar bone, surrounding remodeled secondary osteonal bone.
Plexiform bone occurs when the rate of formation is greatly increased by continually constructing a trabecular network on the surface and filling in the gaps. The result is a mixture of woven bone (the trabeculae) and lamellar bone (the filled-in spaces). Plexiform bone contains rectilinear residual vascular spaces, which often produce a “brick wall” appearance. Figure 2.8 contains brightfield and polarized light photomicrographs of this structure. It typically occurs in large, fast-growing animals like cows. Racehorses, which put enormous stress on some of their bones, have a similar kind of bone that may have exceptional fatigue resistance (Stover et al. 1992).


Figure 2.8
(a) Photomicrograph of plexiform bone. Vascular channels run between the blocks of bone. (b) In polarized light, one can appreciate the structure of the plexiform bone, with non-lamellar bone forming the core (which is dark in this image), surrounded by lighter primary lamellar bone. This bone is from a cow. Orig. mag. ×5.
Secondary bone results from the resorption of existing bone and its more or less immediate replacement by new, lamellar bone. This process is known as remodeling (see Chap. 3). In compact bone, secondary tissue consists of cylindrical structures known as secondary osteons or Haversian systems (Fig. 2.9a). These are about 200 μm in diameter, although this varies with age in predictable ways (Currey 1964; Jowsey 1966; Martin et al. 1980), and consist of about 16–20 cylindrical concentric lamellae surrounding a central Haversian canal. Osteons are about 1–10 mm long and in the human femur they run at an angle of 11–17° with respect to the long axis of the bone (Cohen and Harris 1958). The boundary between the osteon and the surrounding bone is known as the cement line. It is composed largely of glycosaminoglycans and can be either poorly mineralized (Schaffler et al. 1987) or highly mineralized (Skedros et al. 2005). The cement line can act as a weak boundary that traps cracks to slow crack growth in cortical tissue (Burr et al. 1988). The weak interface between the osteon and the rest of the bone matrix increases tissue toughness and fatigue life. A cement line with low mineralization can be a viscous material that may relieve locally high shear stresses. The low shear stiffness reduces the ability of a cement line to transmit energy to a growing crack. Displacement (creep) of cement lines has been observed in human bone over long periods of torsional loading (Lakes and Saha 1979). In adult humans, most compact bone is entirely composed of secondary bone, which may include whole osteons and the remnants of older osteons that have been partially resorbed (interstitial bone). Remodeling of individual trabeculae within cancellous bone, which occurs frequently in both children and adults, rarely produces a complete osteon because the 200 μm diameter osteon is too large to fit within most trabecula of average diameter 100–150 μm. Instead, hemi-osteons are formed (Fig. 2.9b). One surface of the hemi-osteon borders the marrow cavity, rather than a Haversian canal, but the remaining surface is separated from older bone within the trabecula by a cement line just as whole osteons are separated from their surroundings in compact bone.


Figure 2.9
(a) Higher magnification image in polarized light of remodeled secondary osteonal bone, taken from a chimpanzee femur. (b) In trabecular bone, new packets of bone are often formed from the surface and do not create an entire osteonal structure. The “half osteons” are termed hemi-osteons. One can also see complete secondary osteons within the individual trabeculae.
2.4 The Collagen-Mineral Composition of Bone
At the ultrastructural level, bone is composed of organic and mineralized components, mainly consisting of a matrix of cross-linked type I collagen mineralized with nanocrystalline, carbonated apatite. About 65 % of bone by weight is mineral, and 20–25 % is collagen, mostly Type I. Although bone is a hard and stiff tissue, water composes about 10 % of its structure and is essential to normal mechanical properties. Non-collagenous proteins make up the remainder, only about 1–2 % of the bone, but have vital regulatory and mechanical functions that belie their relatively minor contribution to bone mass (Table 2.1).
Table 2.1
Composition of whole bone by weight dogs
Component | Site or specific molecule | Volume (%) |
---|---|---|
Water, 10 % | Bound | 60–80a |
Free | 20–40a | |
Organic matrix, 25 % | Collagen | 90 |
Non-collagenous proteins | 10 | |
Apatite mineral, 65 % | In gaps between collagen ends | 28 |
Intrafibrillar | 58 | |
Interfibrillar | 14 |
Collagen is a structural protein (molecule), widely distributed throughout the animal kingdom that can spontaneously organize itself into strong fibers. Several dozen types of collagen have now been identified, although only a few of these are found in bone. The predominant fibrillar collagen in bone is Type I, which is also found in tendons, ligaments and skin. Type I collagen is arranged in a triple helix that aggregates into a periodic arrangement of parallel molecules spaced in a quarter-staggered array with separations of ~67 nm between their ends to form collagen fibrils of about 150 nm (Fig. 2.10). The mean diameter of collagen fibrils in osteoporotic bone is less than in healthy bone, and this may increase bone’s fragility. Variations in spacing may also accompany certain diseases affecting collagen formation, such as osteogenesis imperfect (Wallace et al. 2011).


Figure 2.10
The structure of a collagen fiber. Note the arrangement of the mineral and water within the fibrils.
Chemical bonds called cross-links form bridges between the collagen molecules, stiffening the fibrils and fibers. Immature divalent cross-links are converted fairly rapidly (within weeks of bone deposition) to mature, or irreducible, trivalent cross-links by enzymatic processes. Cross-links created by non-enzymatic processes can also be formed. Non-enzymatic reactions between sugars and proteins are common. After a series of Maillard reactions the products are called advanced glycation endproducts (AGEs). Some of the AGEs create collagen cross-links that stiffen collagen fibers in a manner similar to normal enzymatic cross-links.
Small amounts of Types III and V collagen can be found in the peri-lacunar region of the osteocytes, and possibly contribute to the organization of the extracellular matrix and the construction of the Type I collagen fiber itself (Olsen 1995).
The amount and maturity of the Type I collagen in bone, and the manner in which it is cross-linked, can have a profound effect on strength, stiffness and energy to fracture of bone tissue. Lower collagen content is a good predictor of reduced energy to fracture (Ding et al. 1997). Increased density of mature, trivalent pyridinoline and deoxypyridinoline cross-links increases the compressive strength and stiffness of bone (Bailey et al. 1992; Banse et al. 2002; Lees et al. 1990; Oxlund et al. 1996), but increases in these natural cross-links have not been demonstrated to change bone’s fracture energy or ductility (Zioupos et al. 1999; Hernandez et al. 2005; Keaveny et al. 1994; Wang et al. 2002). There is a smaller proportion of immature divalent cross-links in newly formed bone from individuals with osteoporosis (Bailey and Knott 1999), which could increase bone fragility and decrease bending strength.
Non-enzymatic Maillard reactions can form AGEs that cross-link the collagen and reduce bone toughness by reducing ultimate strain and by post-yield deformation. They reduce collagen fibril diameter by dehydration, which could also contribute to increased fragility. AGEs naturally accumulate in bone with age. Bone from individuals over 70 years old has three times more non-enzymatic cross-links than bone from those less than 50 years. AGEs are found in abundance in diabetic bone. Bone from these individuals is more fragile and more likely to break, even in the face of normal or elevated bone mineral density.
Mineral in bone consists almost entirely of highly-substituted, poorly crystalline apatite mineral (Ca10PO4)6(OH)2 that nucleates within the spaces between the ends of the collagen fibrils, known as hole-zones, as well as in ~35 nm gap zones known as pores that run longitudinally between the fibrils. The individual crystals are rods or plates with hexagonal symmetry, measuring about 50 × 50 × 400 Å (1 μm = 10,000 Å). As bone tissue matures, the mineral crystals grow, become more plate-like and orient themselves parallel to one another and to the collagen fibrils. Therefore the measured average size of mineral crystals is highly dependent on tissue age. In both cancellous and cortical bone, the c-axis, or long axis, of the mineral and collagen aligns with the longitudinal axis of the structure, i.e. the trabecula or the osteon, respectively. When bone is fully mineralized, the crystals are sufficiently close to one another that removal of the organic matrix by ashing or by chemical means leaves behind a solid piece of material rather than a pile of nanocrystalline “dust.” It is unknown whether the crystals actually merge together into a single continuous phase such as in a stony material like granite or are cemented together like sandstone, but their mechanical behavior in fully mineralized tissue seems to be similar to a continuous polycrystalline solid.
The hydroxyapatite found in bone contains many ionic substitutions (e.g. strontium, lead, carbonate, fluoride). These substitutions are governed by the composition of the body fluids and in turn affect the solubility and material behavior of the bone mineral. As bone matures, mineral crystal size and perfection increases due to changes in ion substitutions and mineral stoichiometry (Miller et al. 2001). These can substantially alter the hardness and brittleness of bone in either a positive or a negative manner. Substituting carbonate for the phosphate in hydroxyapatite makes the crystal more soluble, reduces the stability of the mineral lattice and increases fragility. Different cation and anion substitutions in the apatite lattice, can in some cases alter the mechanical properties of bone and in others affect the activity of osteoblasts and osteoclasts. Although not a strict substitution due to size constraints, citrate binding on the surface of the apatite crystal lattice prevents further crystal growth, leading to thinner nanocrystals that may render the overall structure more resistant to crack propagation (Hu et al. 2011).
It is clear that there is a positive correlation between tissue mineralization and stiffness, but also an inverse relationship between these and toughness (Currey 2004). As the mineral in bone increases, the tissue becomes stiffer, stronger and more brittle. The stiffer tissue deforms less under load and when the bone begins to fail, cracks will propagate with less energy than in less mineralized bone. Thus, a higher level of mineralization makes it more difficult to start a crack in the bone (it is stronger) but once a crack starts it is more likely to propagate and break the bone. Lower mineralization may therefore be beneficial, but only to a point; under-mineralized bones, such as occur in rickets, are weak and readily break. Women who have osteoporotic fractures tend to have bones that are either in the highest or the lowest quartile of the mineralization spectrum (Ciarelli et al. 2003).
There are many non-collagenous proteins (NCPs) and polysaccharides (sugar polymers) that have different functions in regulating the assembly and mineralization of the bone matrix. Non-collagenous molecules can be divided into several large groups that include the proteoglycans (versican, heparin sulfate, biglycan, and decorin); the glycoproteins (alkaline phosphatase, thrombospondin 1 and 2, fibronectin, vitronectin); the SIBLING proteins (osteopontin, bone sialoprotein, Dmp-1, MEPE); and several other ubiquitous and very important proteins, including osteocalcin and osteonectin. The proteoglycans have variable spatial locations with versican expressed highly in osteoid and prevalent during skeletal development. Decorin and biglycan regulate the construction of collagen fibers, and their mineralization, and act in the differentiation of bone forming cells (osteoblasts). The glycoproteins are active in bone formation and mineralization, but are also important during development in the early stages of bone formation and cell proliferation. The SIBLING family of proteins regulate bone mineralization, and several of them (Dmp-1, MEPE) are expressed highly by osteocytes. Osteocalcin and osteonectin (also called SPARC) modify mineral deposition. Osteocalcin is a marker of bone formation (or turnover), whereas osteonectin plays a role in osteoblast proliferation and helps to regulate growth factors in bone.
Box 2.3 The Interaction of Collagen and Mineral Plays a Role in Mechanical Behavior
The interaction of collagen and mineral adds another level of complexity to the mechanical properties of bone. Although collagen supports most of the matrix strain in tension (Gupta et al. 2006), there is a coupled deformation between collagen and mineral. Tensile strain is distributed through an elongation of collagen fibrils and shear deformation of the interfibrillar matrix (Gupta et al. 2006). This protects the hydroxyapatite, which is by itself brittle, and doubles its load-carrying capacity (Gupta et al. 2006) while evenly distributing the forces and reducing stress throughout the matrix (Fratzl et al. 1994; Gao et al. 2003). Smaller collagen fibers oriented around osteocytes alter local strains at the nano- and micrometer level, diverting cracks away from living cells, and preserving them (Ascenzi et al. 2008). The orientation of mineral crystallites also changes the strain environment at the nano-scale (Giri et al. 2008). Thus, the mechanical properties of the collagen and mineral composite, and the viability of bone cells, are enhanced through coupling and load transfer around cellular lacunae and other discontinuities in bone.
Some of the water in the calcified bone matrix is free, and some is bound to other molecules. This water increases ductility and toughness of the bone by allowing sliding between the collagen fibrils and the mineral components (Nyman et al. 2008; Buehler 2007; Fritsch et al. 2009). The mineralization of osteoid (the organic portion of extracellular bone) displaces part of its water, which increases stiffness but decreases the ductility of the tissue. Table 2.1 gives the approximate composition of bone tissue by weight in dogs, measured in primary or secondary lamellar bone several months after formation.
2.4.1 Quantitative Analyses of Bone Composition
We have seen that bone contains three primary constituents: mineral, an organic matrix that is largely collagen, and water. Clearly, these three substances have distinctive physical properties that contribute to the overall mechanical behavior of the tissues they comprise. Architectural organization and substrate interactions are also important contributors to skeletal performance but the relative masses of bone’s three primary constituents are a good indicator of bone health and enable a reasonable first approximation of its intrinsic strength and stiffness.
Simple gravimetric analysis is a time-honored method of determining the mineral, organic and water content of extracted bones. In this method bone specimens are precisely weighed before and after drying in an oven at 100 °C to determine wet weight, dry weight and percent water. The specimen is then placed in a muffle furnace and heated to over 600 °C which volatizes all organic constituents and removes any remaining bound water leaving the ash mass which is weighed to determine the mineral component of the tissue. The organic content (predominately collagen) and ash content (mineral) are then determined as a percentage of the original wet weight. Tissue mineralization or ash fraction is calculated as the percentage of ash weight to dry weight. The ash fraction is independent of bone porosity and is an excellent measure of tissue mineralization, which varies by species, skeletal site, age and disease. Collagen content can be measured as the difference between total dry weight and ash weight, divided by dry weight. The gravimetric method can be extended to measure carbonate content by heating specimens to above 900 °C, the temperature at which incorporated carbonate decomposes to CO2.
Gravimetric analysis yields good quantitative information about the major constituents of bone but it cannot measure the quality of collagen or mineral in the sample, nor can it provide architectural information related to bone density and porosity. The average weight density of the mineralized matrix (ρbone) can be determined in the laboratory using Archimedes law of buoyancy using a fluid of known weight density (ρfluid = 1 g/cm3 for distilled water; Eq. 2.1) provided that all marrow elements and fat are removed beforehand.

The density of either cortical or trabecular bone specimens can be measured using this approach, but completely removing marrow elements and fat from highly porous trabecular samples is difficult. Apparent density, the weight of the sample divided by its total overall volume (typically a machined cube or cylinder), including the pores and spaces within, is a far more common measure for trabecular samples. Once the apparent density is known the porosity (P) of the sample can be determined using the following equation:

where ρapparent is the apparent density of the sample and ρbone is the density of the bone tissue in the sample as determined directly using Archimedes principle or another technique. A generally accepted value of 2.0 g/ml is frequently substituted for direct measurements of ρbone, thereby allowing for easily obtained estimates of porosity.

(2.1)

(2.2)
The porosity (P) of a bone sample is simply the percentage of total sample volume not occupied by bone tissue. Conversely, the bone volume fraction (BV/TV) of a sample is the percentage of volume occupied by bone matrix. The material comprising the bone volume fraction is often referred to as bone tissue to distinguish it from the term “bone,” which usually refers to a region large enough to contain Haversian canals, marrow spaces, or other voids. Cancellous bone has been somewhat arbitrarily defined as having porosity greater than 0.5, with free space largely occupied by marrow elements; in cortical bone, porosity is less than 0.5 and space is defined by Haversian and Volkmann’s canals and resorption cavities created by osteoclasts. For practical reasons, the smallest voids in bone, consisting of octeocyte lacunae and canaliculi, have traditionally been included in the bone volume fraction rather than the porosity fraction.
The foregoing describes weight-based measures of bone volume, density and porosity. Another traditional method of analysis employs histologic sections and the principles of stereology. Before the advent of automated procedures this approach typically entailed overlaying a two-dimensional histologic image of a bone section with a series of lines or an array of points and measuring the length of line passing through, or the number of points landing on, bone or void space. When enough sections are sampled the approach yields accurate estimates of bone composition because the ratio of bone tissue to void space remains the same whether assessed by counting points (0D), measuring line lengths (1D), measuring areas (2D) or measuring volumes (3D). Bone volume, bone density and a host of other architectural measures such as bone surface area, trabecular number, thickness and trabecular spacing are now easily made using newer 3D imaging modalities and automated procedures.
2.4.2 Three Dimensional Imaging of Bone Using High Resolution Computed Tomography (microCT)
A bone can change shape both from the outside and the inside as a result of age, diet, disease, fracture and from many other causes. Today, there are many ways to image the shape of a bone in a person or animal without harming them. X-Ray Computed Tomography (CT or “Cat Scan”) is often the best method for seeing bones inside the body. The machines used in hospitals ordinarily have the resolution to image clearly objects down to about a millimeter, which is outstanding for many medical purposes but insufficient to see trabeculae, osteons, Volkmann’s canals (blood vessels) and other microanatomy in bones that contribute to its health and strength.
There now are many commercially available CT scanners for small specimens that have better than one millimeter resolution. The highest resolution machines are often called “X-Ray Microcomputed Tomography” scanners or “MicroCT” scanners for short. For resolution on the order of 35–50 μm there are machines that can be used to image the bones of small animals such as mice or rats while they are alive. For finer resolutions on the order of 1–10 μm the required x-ray doses are dangerous to life making high resolution scanning usually restricted to dead or nonliving specimens.
The three dimensional (3D) images obtained from microCT (Fig. 2.11) are used to understand bone microarchitecture in many ways. One method is to use computer assisted image processing to measure bone density or connectivity and to count trabeculae or other features directly from the image (Hildebrand et al. 1999). In the past, measurement of the interior of a bone specimen was done by physically sectioning the bone, mounting the thin histologic bone specimens onto microscope slides and using stereometric analyses (Parfitt et al. 1987; Russ and DeHoff 2000) to estimate 3D information from the 2D microscopic images. MicroCT scanning and image analysis has eliminated much of the need to use bone histology for measuring the interior microanatomy of bone, although histology is still necessary to image bone cells since microCT cannot resolve them adequately. Histology is, of course, still necessary to measure cell-level information such as gene expression and protein distribution, or to quantify dynamic bone remodeling.


Figure 2.11
Micro-computed tomography “slice” from a specimen of human vertebral cancellous bone (50 μm resolution). The lightest colors are mineralized bone tissue, the gray is bone marrow and the darkest is air.
A powerful tool for understanding how bone microstructure contributes to tissue strength and stiffness is to convert the 3D microCT image into a finite element stress analysis model (Fig. 2.12). Finite element models of bone tissue built in this way are much better at predicting the mechanical properties of bone tissue than are simpler models that don’t include microanatomy (Hou et al. 1998; Homminga et al. 2002; Zauel et al. 2006). Prior to the invention of high resolution microCT finite element modeling of trabecular bone in 3D was extraordinarily time consuming or impossible. Now, however, stress analysis is a normal part of research into what makes bones weak or strong. With continued technological development, higher resolution CT scanners may become available to hospital patients and some of the insights from microCT could become useful to predicting the risk of bone fractures for individual patients.
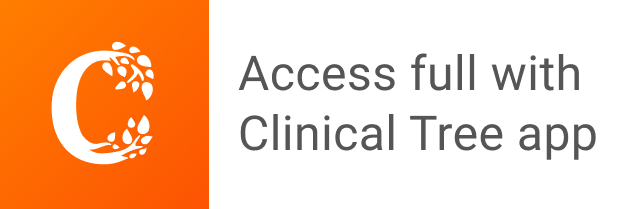