Fig. 1.1
The mouse noninvasive tibia axial loading model. (a) Overview of the experimental design. (b) Loading-related osteogenesis labeled by calcein green on the first day of loading and alizarin red on the last day of loading and loading-induced strain distribution by finite element analysis. (c) Relationship between peak dynamic load and strain on the center of the lateral surface in the right proximal/middle tibiae, where predominant osteogenesis can be induced, in 17-week-old mice with right sciatic neurectomy. (d) Representative strain recording, induced by a peak dynamic load of 12 N, on the center of the lateral surface in the right proximal/middle tibiae of 17-week-old mice with right sciatic neurectomy (Adapted from Sugiyama et al. [41])
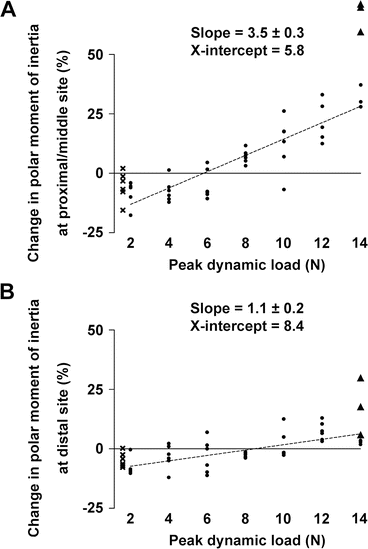
Fig. 1.2
Relationship between peak dynamic load and the change ([right–left]/left) in polar moment of inertia (J), a parameter of structural bone strength, in the tibiae of 20-week-old mice that received right sciatic neurectomy and axial loading in the right tibia. (a) Proximal/middle site. (b) Distal site. Best-fit and SE values of slope and best-fit values of x-intercept are shown. ● = mice with no apparent woven bone formation, X = mice without external dynamic loading, ▲ = mice with apparent woven bone formation. Note that X (n = 6) and ▲ (n = 3) were excluded for the multilevel regression analyses (Adapted from Sugiyama et al. [41])
Notably, the above continuous response in the mechanostat is consistent with the results in humans [52] as well as other experimental models [6, 53, 54]. This is entirely compatible with studies in which bones under normal physical activity are additionally subjected to mechanical loading [20, 55, 56] showing osteogenic responses only above certain levels of peak strain magnitude, because artificial (external) loading would stimulate the bone only when this stimulus exceeds that already derived from natural (internal) loading.
1.2.1.2 Local Control
Most in vivo experiments of external mechanical loading use animals in which artificial loads are applied to the bones on one side, and the osteogenic responses in the loaded bones have been generally compared with those in the non-loaded contralateral pair. For this approach to be valid, it is essential that the adaptive response of the loaded bones is confined to those bones and does not influence their contralateral controls. However, this assumption has been challenged by recent reports showing the systemic effects of mechanical loading [57–59].
This possibility was investigated [38]. In brief, skeletally mature female C57BL/6 mice were randomly assigned to one of the following three groups; all groups were treated with isoflurane anesthesia three times a week for 2 weeks (approximately 7 min/day). During each anesthetic period, the right tibiae/fibulae in the DYNAMIC + STATIC group were subjected to dynamic loading superimposed upon a static preload to hold the bones. The right tibiae/fibulae in the STATIC group received the static preload alone, while the NOLOAD group received no artificial loading. Bilateral tibiae, fibulae, femora, ulnae, and radii were analyzed by high-resolution μCT and histomorphometry. As a result, the adaptive response in both cortical and trabecular regions of the bones subjected to dynamic loading, even when this response was sufficiently vigorous to stimulate woven bone formation, was confined to the loaded bones and did not involve changes in other bones that are adjacent, contralateral, or remote to them (Figs. 1.3 and 1.4).
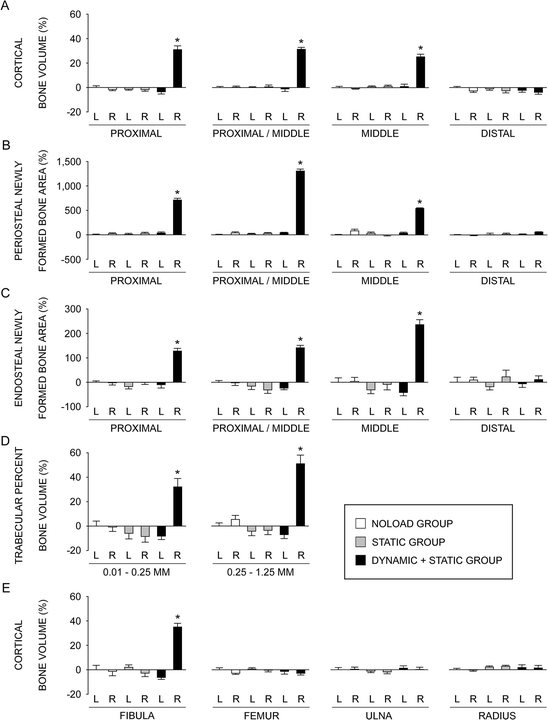
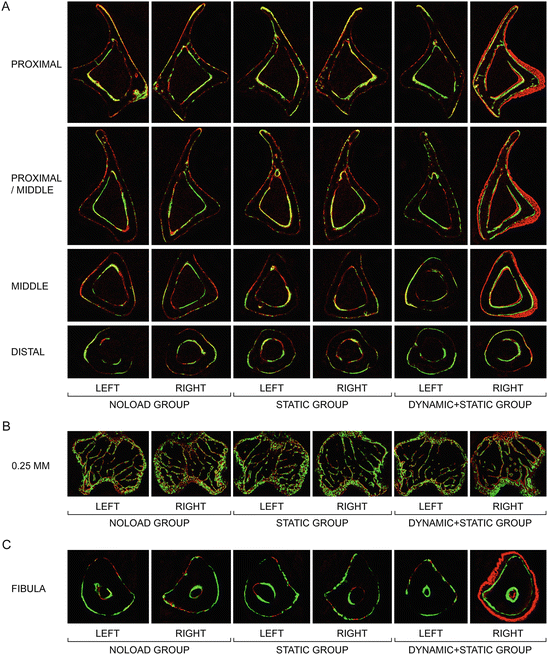
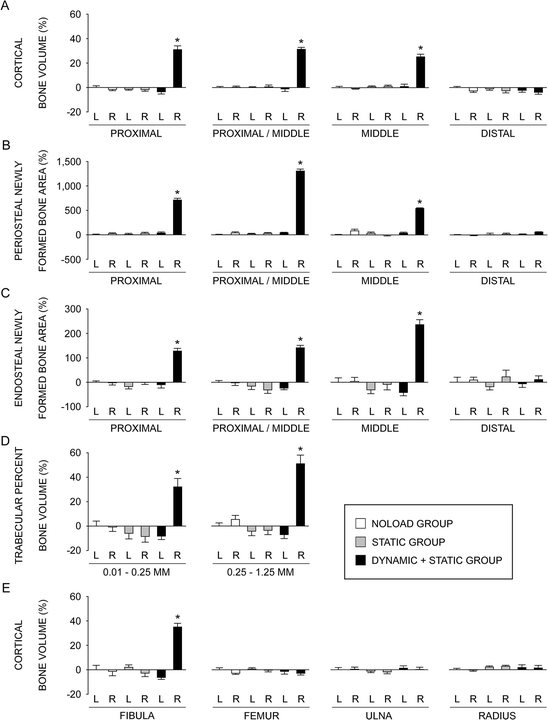
Fig. 1.3
Relative values, analyzed by μCT and histomorphometry, of the left and right bones in the NOLOAD, STATIC, and DYNAMIC + STATIC groups compared to the left bones in the NOLOAD group. L left, R right. (a) Cortical bone volume analyzed by μCT at the proximal (25 % of the bone’s length from its proximal end), proximal/middle (37 %), middle (50 %), and distal (75 %) sites of the tibia. (b) Periosteal labels and inter-label bone area, analyzed by histomorphometry, normalized by total cortical bone area at the proximal, proximal/middle, middle, and distal sites of the tibia. (c) Endosteal labels and inter-label bone area, analyzed by histomorphometry, normalized by total cortical bone area at the proximal, proximal/middle, middle, and distal sites of the tibia. (d) Trabecular percent bone volume analyzed by μCT at two sites 0.01–0.25 mm (containing primary spongiosa) and 0.25–1.25 mm (secondary spongiosa) distal to the growth plate in the proximal tibia. (e) Cortical bone volume analyzed by μCT at the middle (50 %) site of the fibula, femur, ulna, and radius. Data are the mean ± SE (n = 6–7). *P < 0.05 versus all other five values by one-way ANOVA followed by a post hoc Bonferroni or Dunnett’s T3 test (Adapted from Sugiyama et al. [38])
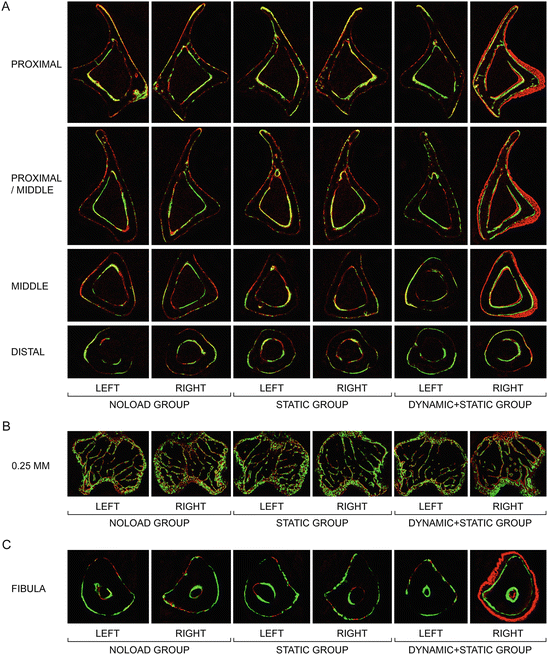
Fig. 1.4
Representative transverse fluorochrome-labeled images. (a) Cortical bone at the proximal (25 % of the bone’s length from its proximal end), proximal/middle (37 %), middle (50 %), and distal (75 %) sites of the tibia. (b) Trabecular bone at the site 0.25 mm distal to the growth plate in the proximal tibia. (c) Cortical bone at the middle (50 %) site of the fibula. Green: calcein label injected on the first day of loading (day 1). Red: alizarin label injected on the last day of loading (day 12) (Adapted from Sugiyama et al. [38])
The above local control in the mechanostat has been confirmed by recent studies [60, 61]. In contrast, the systemic effects of mechanical loading [57–59] might be associated with the loading regimen [38]. Nevertheless, the protocol of mechanical loading should be designed to produce a realistic physiological stimulus capable of stimulating a measurable osteogenic response while avoiding collateral stimulation associated with trauma and interference with blood supply both within the bone and around the loading cups. It is therefore important to note that any loading protocol using the contralateral non-loaded bone as a control can be accepted only after validation of the local control.
1.2.1.3 Osteoporosis Drugs
Parathyroid Hormone
Several in vivo experiments of external loading in rats have shown that intermittent treatment with parathyroid hormone (iPTH) and high-magnitude loading synergistically increase bone formation [62–65]. Although high-impact exercise to increase bone strength would be difficult for older patients with skeletal fragility, iPTH could reduce the loading level necessary to stimulate a loading-related anabolic effect.
An experiment was performed to investigate this concept [37]. In brief, female C57BL/6 mice from 13 to 19 weeks of age were given daily injections of vehicle or iPTH (1–34) at low (20 μg/kg/day), medium (40 μg/kg/day), or high (80 μg/kg/day) dose. For three alternate days per week during the last 2 weeks of this treatment, the tibiae and ulnae on one side were subjected to dynamic axial loading. Two levels of peak load magnitude, one sufficient to engender an osteogenic response and the other insufficient to do so, were applied. The whole tibiae and ulnae were analyzed by high-resolution μCT and histomorphometry. As a result, in the tibia, loading at a level sufficient by itself to stimulate osteogenesis produced an osteogenic response in the low-dose iPTH (1–34)-treated trabecular bone and in the proximal and middle cortical bone treated with all doses of iPTH (1–34). In the ulna, loading at a level that did not by itself stimulate osteogenesis was osteogenic at the distal site when combined with high-dose iPTH (1–34). At both levels of loading, there were synergistic effects in cortical bone volume of the proximal tibia and distal ulna between loading and high-dose iPTH (1–34) (Fig. 1.5). Images of fluorescently labeled bones confirmed that such synergism resulted from increases in both endosteal and periosteal bone formation. No woven bone was induced by iPTH (1–34) or either level of loading alone, whereas the combination of iPTH (1–34) and the sufficient level of loading stimulated woven bone formation on endosteal and periosteal surfaces of the proximal cortex in the tibiae. Consistent with these experimental data, daily treatment with teriparatide could synergistically produce bone gain with physiological levels of mechanical loading in humans [66].
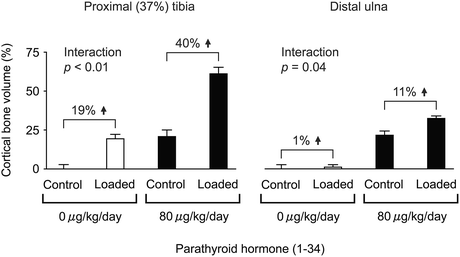
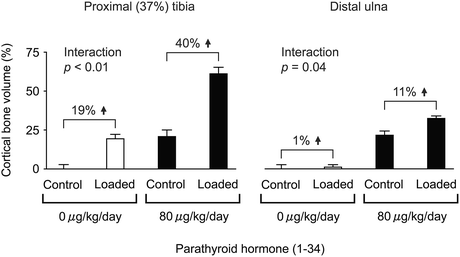
Fig. 1.5
Relative effect of 6 weeks of high-dose iPTH (1–34) and 2 weeks of mechanical loading alone or in combination on cortical bone volume at the proximal (37 %) tibia and distal ulna in 19-week-old female C57BL/6 mice. Levels of peak load: sufficient to engender an osteogenic response in the tibia and insufficient to do so in the ulna. Mean ± SE (n = 5–8). Interaction between high-dose iPTH (1–34) and mechanical loading by two-way ANOVA (Adapted from Sugiyama et al. [37])
Bisphosphonate
The combination effects of bisphosphonates and mechanical loading were studied in a variety of external loading models in rodents. As mentioned earlier, mechanical loading can stimulate bone formation independently of bone resorption [14], and pamidronate did not change osteogenesis caused by loading in the rat tail [15]. Similarly, alendronate, risedronate , and zoledronic acid at clinical doses did not influence periosteal expansion induced by loading in the rat ulna [67]. In contrast, the response of the cortical bone to loading was impaired by zoledronic acid in the mouse tibia [68] and minodronate at higher doses but not the optimal dose for osteoporosis treatment in the rat tibia [69].
An experiment was performed to assess the separate and combined effects of risedronate and mechanical loading on the trabecular and cortical bone [39]. In brief, 17-week-old female C57BL/6 mice were given daily subcutaneous injections of vehicle or risedronate at a dose of 0.15, 1.5, 15, or 150 μg/kg/day for 17 days. From the fourth day of treatment, the right tibiae were subjected to mechanical loading for three alternate days per week for 2 weeks. Trabecular and cortical sites in the tibiae were analyzed by high-resolution μCT and histomorphometry. As a result, in the non-loaded tibiae, treatment with the higher doses of risedronate at 15 or 150 μg/kg/day resulted in higher trabecular bone volume and trabecular number than in vehicle-treated controls, whereas such treatment was associated with no differences in cortical bone volume at any dose. In the loaded tibiae, loading induced increases in trabecular and cortical bone volume compared with contralateral controls primarily through increased trabecular thickness and periosteal expansion, respectively, independently of risedronate treatment. In conclusion, the response to mechanical loading in both trabecular and cortical bone in mice was not impaired by risedronate , even over a 1000-fold dose range (Fig. 1.6). This is consistent with mechanical loading -related bone modeling [14]; formation and resorption occur on different surfaces during bone modeling, and thus, modeling-based bone formation and resorption are not coupled. In considering the optimization of clinical treatments for osteoporosis, it is reassuring that antiresorptive therapy and mechanical loading can exert independent beneficial effects.
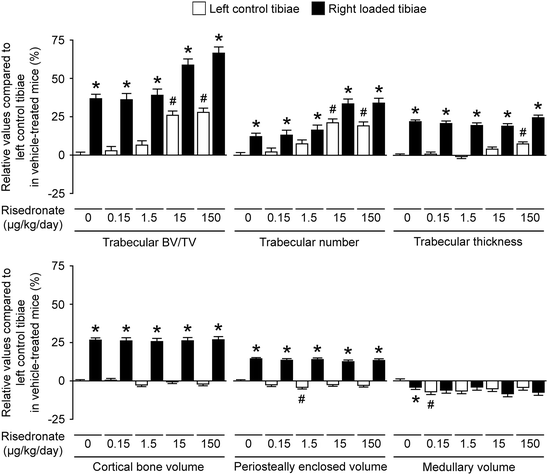
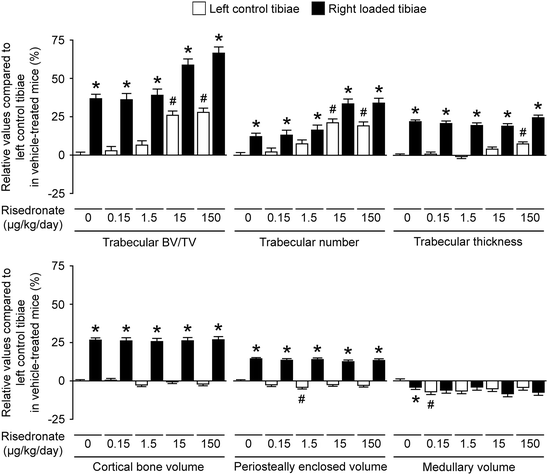
Fig. 1.6
Relative values of trabecular and cortical μCT parameters of the left control and right loaded tibiae in mice treated with vehicle or risedronate at a dose of 0.15, 1.5, 15, or 150 μg/kg/day compared to the left control tibiae in vehicle-treated mice. Values were obtained from mixed model analysis including body weight and are presented as mean ± SE (n = 20 in vehicle treatment and n = 10 in risedronate treatments). # P < 0.05 versus left control tibiae in vehicle-treated mice and *p < 0.05 versus left control tibiae in each treatment with vehicle or risedronate by mixed model analysis followed by Bonferroni adjustment (Adapted from Sugiyama et al. [39])
1.3 The Mechanostat -Based Clinical Perspectives
In addition to other chronic diseases such as hypertension, hypercholesterolemia, and diabetes, a treat-to-target strategy was recently applied in rheumatoid arthritis and has now been discussed in osteoporosis. An important goal of osteoporosis therapy is to achieve normal risk of hip fracture associated with significant morbidity and mortality, but the anti-fracture efficacies of currently approved osteoporosis drugs are limited [70–72]. Here, it is important to note that the human skeleton normally adapts to mechanical environment [52, 73–76].
1.3.1 Limitation of Osteoporosis Therapy
The adult skeleton in humans would continuously respond to change in mechanical environment to maintain resultant strain of the bone [52, 76], while increased bone strength by an osteoporosis drug results in decreased bone strain regardless of suppressing bone resorption or promoting bone formation. This suggests that the effect of osteoporosis therapy is limited by skeletal adaptation to mechanical strain , i.e., the natural homeostatic system in the skeleton (Fig. 1.7) [77, 78], which is consistent with the fact that there exists a powerful effect that returns bone mass to its pretreatment level after the withdrawal of treatment with osteoporosis agents. In addition, this theory can provide a new significant insight into the mechanisms by which vitamin D or warfarin affects the skeleton [79–81].
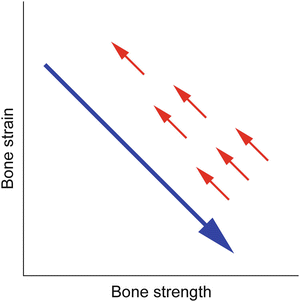
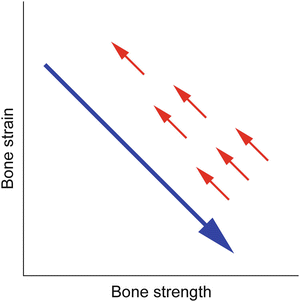
Fig. 1.7
Mechanical strain -related feedback control of bone strength: natural homeostatic system in the skeleton. A long arrow indicates the effect of osteoporosis therapy that increases bone strength and thus decreases bone strain from physical activity, regardless of suppressing bone resorption or promoting bone formation or increasing bone quantity or quality. Short arrows indicate the negative feedback control of bone strength that returns bone strain to its pretreatment level (Adapted from Sugiyama et al. [77])
A strategy to reduce the limitation of osteoporosis therapy is pharmacologically enhancing skeletal response to mechanical stimulation. Advantages of this strategy include increasing bone strength safely in a structural appropriate manner, depending on local mechanical environment at each skeletal site. Among drugs currently approved for the treatment of osteoporosis, only intermittent treatment with parathyroid hormone would have such a possibility; although high-impact exercise to increase bone strength is not easy for older patients with skeletal fragility, teriparatide has been suggested to have a synergistic effect with even low, physiological levels of mechanical loading in animals [37] and humans [66].
There is, however, a disadvantage of the strategy to enhance skeletal response to mechanical stimulation. The skeleton is adapted to the mechanical environment resulting from habitual physical activity, but not to the unusual direction of mechanical force by falls. As a result, the enhancement of bone response to daily physical activity might not efficiently reduce the risk of fall-related hip or non-vertebral fractures. One approach to overcome this disadvantage is to find an agent that has the effect of mechanical strain . For example, it has been shown in animals that the production of sclerostin secreted by osteocytes is increased by skeletal disuse and decreased by skeletal loading [36, 42, 82–84].
1.3.2 Alternative Drugs of Mechanical Strain -Related Stimulus
Consequently, anti-sclerostin antibodies such as romosozumab and blosozumab can be considered as the alternative drugs of mechanical strain -related stimulus [85]. The latest findings include marked modeling-based bone formation by romosozumab in monkeys [86] and rapidly increased bone formation as well as decreased bone resorption by romosozumab [87, 88] and blosozumab [89, 90] in postmenopausal women. In contrast to bone remodeling, modeling-based bone formation and resorption are not coupled, and mechanical stimulation is a natural uncoupling factor that stimulates bone formation and inhibits bone resorption.
In phase 2 studies of postmenopausal women with low areal bone mineral density (BMD), romosozumab and blosozumab markedly increased areal BMD at the lumbar spine and hip dose-dependently, but areal BMD at the one-third radius was not changed even by the highest dose of romosozumab [88] or blosozumab [90]. Experimental evidence that the production of sclerostin secreted by osteocytes is increased by skeletal disuse and decreased by skeletal loading [36, 42, 82–84] implies that even the highest doses of romosozumab and blosozumab were not enough for the radius because the levels of sclerostin expression in non-weight-bearing bones such as the radius could be higher than those in weight-bearing bones such as the lumbar spine and hip. Several lines of evidence to support this hypothesis include (1) patients with sclerosteosis due to deficiency of sclerostin have higher areal BMD at the radius as well as the lumbar spine and hip [91] and (2) appropriate doses of anti-sclerostin antibodies effectively increase bone mass in animals with skeletal disuse or unloading [92, 93]. If correct, the highest doses of romosozumab and blosozumab are unlikely to cause unwanted bony overgrowth at non-weight-bearing sites such as the face and skull in postmenopausal women with osteoporosis, while further higher doses of these drugs would be required to improve skeletal fragility in patients with reduced physical activity.
The existence of other mechanotransduction pathways independent of sclerostin [94], however, indicates that treatment with an anti-sclerostin antibody cannot escape from the mechanostat -related limitation of osteoporosis therapy (Fig. 1.7) [77]. In fact, both romosozumab and blosozumab treatments in postmenopausal women with low areal BMD showed that marked changes in circulating bone formation and resorption markers returned to the pretreatment levels within a year despite the continued treatments [88, 90]. This theory is also compatible with the relation between circulating sclerostin and bone mass; sclerostin -related high bone mass in patients with sclerosteosis or van Buchem disease and heterozygous carriers of these diseases is linked to lower levels of circulating sclerostin [95, 96], while circulating sclerostin and bone mass in normal women and men have a positive correlation [97, 98]. The discrepancy suggests that higher bone mass associated with other mechanotransduction pathways independent of sclerostin would cause lower mechanical strain in the skeleton and thus could result in compensatory higher sclerostin production according to the mechanostat .
1.3.3 Bone Quality Associated with Mineral Versus Collagen
Fall-related fracture occurs if the energy from the fall is higher than that the bone can absorb. Force-displacement curve obtained from a biomechanical test shows that energy absorption, the area under the curve, represents bone fragility and an ideal strategy for the improvement of bone fragility is to increase both of the force and displacement at failure [99] (Fig. 1.8a).
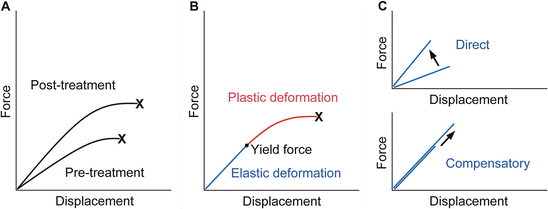
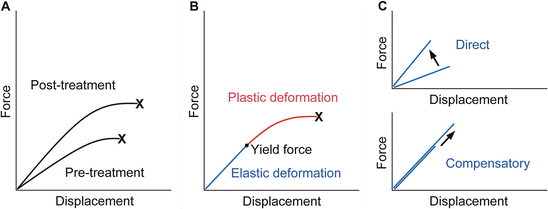
Fig. 1.8
Force-displacement curve of a bone. (a) Treatment with an ideal osteoporosis drug improves bone fragility by increasing both the force and displacement at failure. X denotes fracture. (b) The curve would consist of the pre-yield “elastic” deformation associated with mineral and the post-yield “plastic” deformation associated with collagen. Consequently, mechanical strain -related feedback control, the mechanostat , could work against mineral-related, but not collagen-related, impairment of bone quality . X denotes fracture. (c) The pre-yield “elastic” deformation can be modified by osteoporosis therapy that directly enhances the response to mechanical loading and increases the slope of the curve (upper) or lowers mineral-related bone quality and results in compensatory bone gain by the mechanostat to maintain the slope of the curve (lower). Note that, in the latter case, the yield force can be increased if compensated efficiently (Adapted from Sugiyama et al. [78])
From a material point of view, stiffness and toughness of bone tissue generally depend on mineral and collagen, respectively [100]. There is a yield force at which a bone begins to deform plastically, and mechanical strain from normal physical activity would be linked to the pre-yield “elastic” deformation associated with mineral, but not to the post-yield “plastic” deformation associated with collagen (Fig. 1.8b). Consequently, mechanical strain -related feedback control could compensate mineral-related, but not collagen-related, impairment of bone quality to maintain “elastic” deformation [78]. Indeed, this theory is compatible with clinical data relating to bone quality. Examples of the mechanostat -based compensation for mineral-related impairment of bone quality would include rickets/osteomalacia and use of warfarin [77, 79–81], while the impairment of bone quality associated with collagen cross-links significantly contributes to skeletal fragility in diabetes [101–103].
Finally, it is possible to speculate that daily treatment with teriparatide improves bone fragility at the hip through the mechanostat -based “modeling-related direct” and “remodeling-related compensatory” mechanisms (Fig. 1.8c). The enhancement of skeletal response to mechanical loading [37, 62–66] would result in the former effect. In contrast, a decrease in the degree of mineralization after the treatment [104] might act to improve bone fragility if compensated efficiently, because compensatory bone gain by the mechanostat to maintain the pre-yield “elastic” deformation could increase the yield force at which a bone begins to deform plastically and thus the energy that the bone can absorb. This possibility is supported by histomorphometric data showing that 1 or 2 years of the treatment results in increases in modeling- and remodeling-based bone formation [105], because the mechanostat suggests that the former “modeling-related direct” effect does not continue for a long time [77].
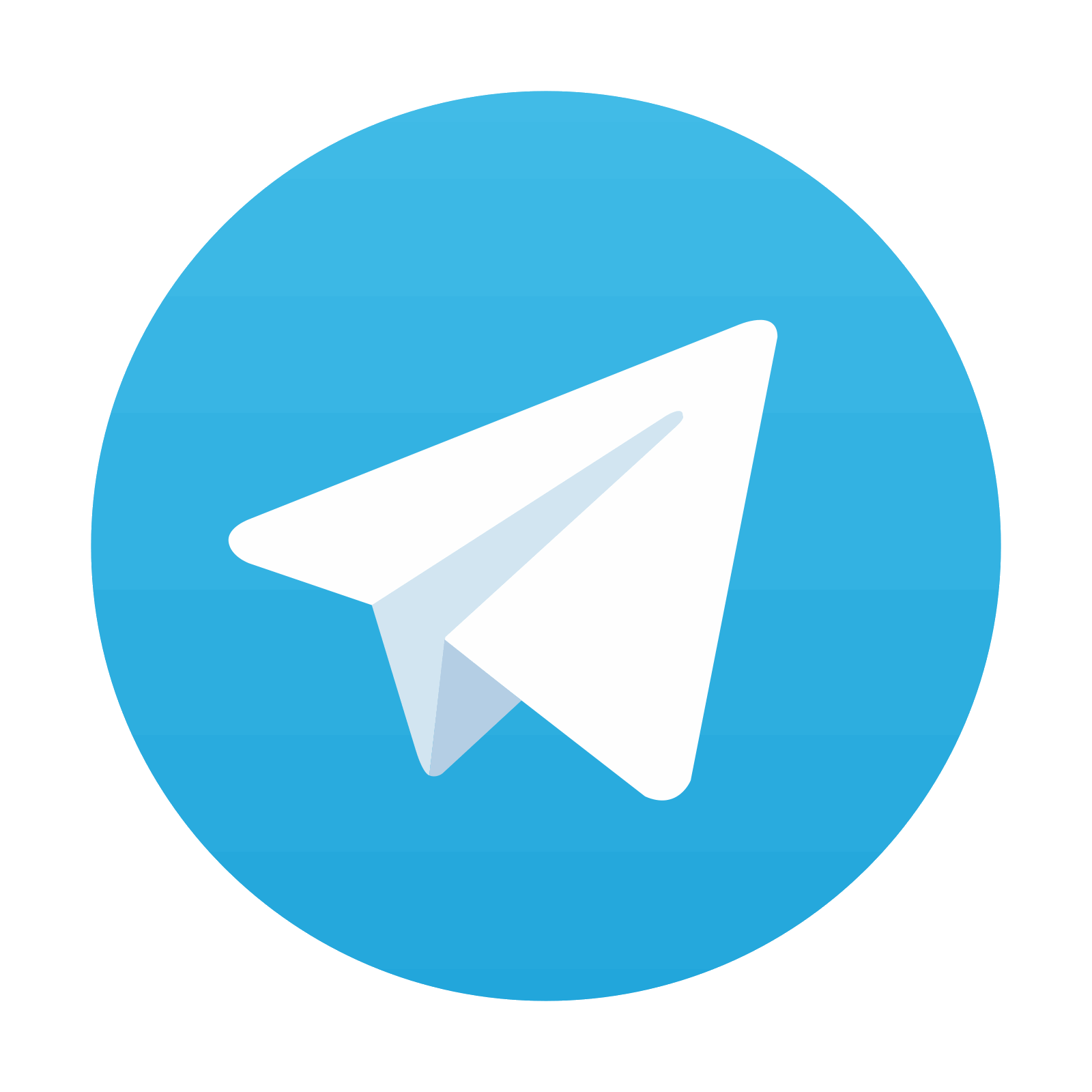
Stay updated, free articles. Join our Telegram channel
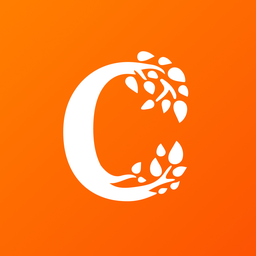
Full access? Get Clinical Tree
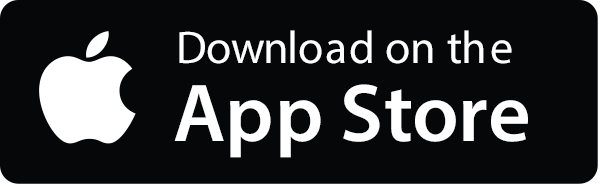
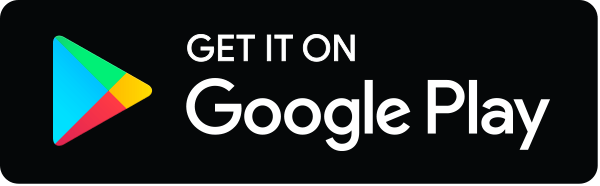