(21.1)

(21.2)
Three other factors affect the force a muscle functionally produces. First, the interdigitated structure of sarcomeres means that there is a varying degree of overlap between the actin and myosin molecules. At its optimum length (L0), all of the available binding sites are available and the sarcomere can generate its maximum force. At both shorter and longer lengths, progressively fewer binding sites are available and a sarcomere’s force-generating capacity progressively diminishes (see active tension curve in Fig. 21.1a).
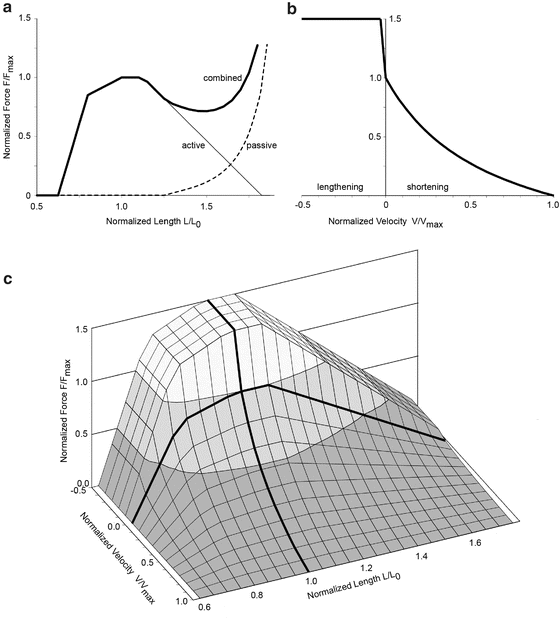
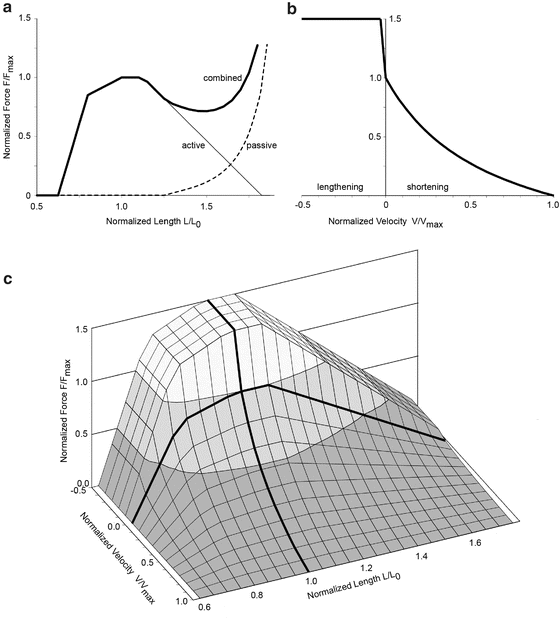
Fig. 21.1
Force, length and velocity relationships for muscle. (a) Normalized force curve showing active (thin line), passive (dashed line) and combined force (thick line) in maximally activated muscle as a function of the normalized length. (b) Normalized force as a function of the normalized shortening/lengthening velocity in maximally activated muscle. (c) Surface representing the normalized force as a function of both normalized length and normalized velocity for maximally activated muscle. Lower levels of activation will produce forces below the surface. Fmax, maximum force; L0, optimum length; and Vmax, maximum shortening velocity
Second, the connective tissues within the muscle generate passive resistance to lengthening similar to any tissue placed in tension (see passive tension curve in Fig. 21.1a). The force generating properties depicted in Fig. 21.1a are for isometric conditions, i.e. when muscle fiber length remains constant. Note that muscle fibers in a contracting muscle spanning a fixed joint angle actually shorten due to lengthening of the tendon, and therefore a fixed joint angle is not truly isometric from the muscle fiber’s perspective.
Third, the speed and direction of a muscle’s contraction can attenuate or amplify a muscle’s force. The chemical processes at the binding sites limit the speed that a muscle can shorten, with the force varying inversely with shortening velocity (Fig. 21.1b). The maximum shortening velocity (Vmax) is often taken to be 10 L0/s [17], however others have reported maximum shortening velocities between 1 and 2 L0/s [18] and variations in maximum shortening velocity with activation level [19]. In contrast to shortening, a lengthening muscle can generate a force greater than its peak isometric force (Fig. 21.1b). This amplification occurs because the force to physically break the bond between the myosin and actin binding site is greater than the force the myosin molecule can generate during the conformational change it undergoes while actively contracting.
The net effect of these length and velocity dependencies is the Force-Length-Velocity (FLV) curve shown in Fig. 21.1c. This normalized surface is used to scale the force values obtained from Eq. 21.1. Techniques for implementing these properties of muscle into computational models can be found in Zajac [17].
Separation of muscle force into active and passive components is a simplification, and more recent work suggests that the passive force component depends on the level of muscle activation [20] and on the muscle contractile history [21].
21.1.3 Muscle Activation Timing
Muscles must be active to generate large forces over their normal operating lengths. In the context of their potential to affect accidental injuries, this means that muscles must be either active before impact, i.e. pre-impact bracing, or activated rapidly during or immediately following impact. There is no debate that a braced muscle is active during impact and can thus alter the forces applied to other tissues during impact; however, there has been debate whether an initially relaxed muscle can be reflexively activated by an impact and then generate sufficient force to affect an injury caused by the impact. Also encapsulated in this latter debate is whether a braced muscle’s force can further increase early enough to affect injury.
Reflex muscle activation can be viewed as three sequential steps (Fig. 21.2). The first step precedes muscle activation and is the interval between the onset of a stimulus and the onset of electrical activity in a muscle. This time interval, called the reflex time, is governed by properties of the stimulus, the sensory organs and the sensorimotor pathways involved. The second step is the time between the onset of electrical activity in the muscle and the onset of muscle force. This time interval, called the electromechanical delay (EMD), is a function of muscle and tendon properties, and is sensitive to how it is measured. The final step is the time between the onset of muscle force and peak muscle force.
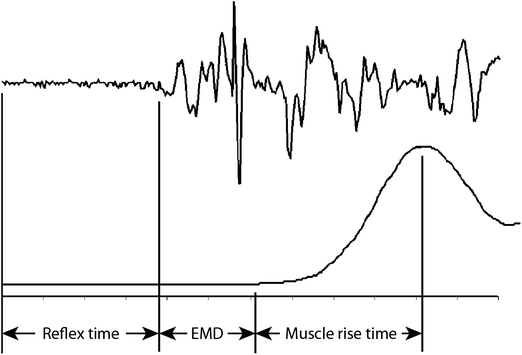
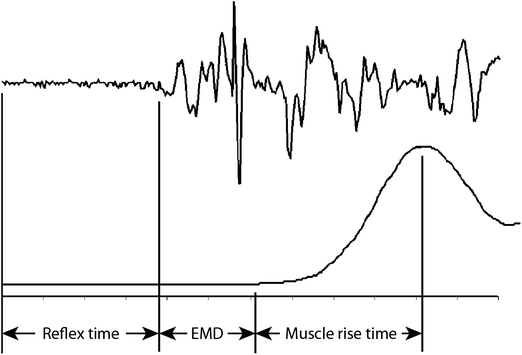
Fig. 21.2
Schematics showing the relationship between electrical activity in a muscle (upper trace) and the production of force by that muscle (lower trace). The stimulus triggering the muscle response occurs at the start of the traces
The reflex times for many axial and appendicular muscles vary from 55 to 100 ms for stimuli varying from a rear-end car crash, supine free fall and acoustic startle [22–25]. These reflex times are shorter than voluntary onset latencies, which, for comparison, are about 105–110 ms in the sternocleidomastoid muscle [26, 27] and 128–130 ms in the tibialis anterior and soleus muscles [28].
Reflex times can shorten slightly with increasing stimulus intensity [23], but there is a minimum reflex time below which increases in stimulus intensity have no further shortening effect. This floor is set by physical constraints like nerve conduction velocities and synapse times. Reflex times can also be shorter when multiple sensory modalities are stimulated. For instance, neck muscle onset latencies during a rear-end collision without noise were about 15 ms longer than when the same collision pulse was presented simultaneously with a loud tone [29]. Onset latencies in the neck muscles are also slightly shorter in females than males [23, 25].
EMD times between 13 and 95 ms have been reported in the literature [30–32]. The large range stems from different ways of measuring its endpoint. Some authors used the onset of movement (video or contact release), which results in long EMD times, whereas others have used the onset of acceleration, which can be detected earlier than the onset of movement and thus results in shorter EMD times. Corcos et al. [33] showed that minimizing measurement-induced artifact by using an accelerometer mounted over a bony prominence yielded EMD times of 13.2 ± 1.1 ms.
Muscle rise times are governed by the intensity of the contraction and the inertia, damping and stiffness of the system the muscle is attempting to move or react against. As a result, there is no estimate of muscle rise time that can be universally applied. However, if the delay between peak electromyographic activity and peak force is similar to the delay between the onset of electromyographic activity and the onset of acceleration, then the rise time of the EMG signal may be a good surrogate for the muscle rise time in a particular situation. During low severity rear-end impacts, the time between sternocleidomastoid (SCM) muscle onset and peak activity (measured using a root mean square of the electromyographic signal) is about 35–43 ms [25, 34].
21.1.4 Muscle Mechanical Properties
Muscle is a difficult tissue to characterize from a mechanical perspective. In addition to being sensitive to loading rates like other soft tissues in the human body, muscle’s highly organized structure creates large anisotropy and its contractile ability results in different properties at different levels of contraction.
Numerous experiments have shown that passive muscle is an anisotropic, highly non-linear viscoelastic material [35–37]. At low strain rates of 0.0005 s−1 in fresh porcine muscle, compression across the fiber direction yielded stresses that were 2–2.5 times higher than those along the fiber direction at 30 % strain [36]. As strain rates increased from 0.0005 to 0.10 s−1, the stress at 30 % strain along the fibers increased more rapidly than across the fibers, ultimately reaching 1.3 times the stress across the fibers at 0.10 s−1 [37].
At compressive strain rates of 700–3,700 s−1, passive bovine muscle was again highly rate sensitive [38–40]. Van Sligtenhorst et al. [40] reported no significant difference in the response between loading along and transverse to the fiber direction at rates of 700–1,250 s−1, although greater difficulty in producing the transverse specimens and greater variability in their results may have contributed to this finding. Song et al. [39] on the other hand reported greater strain rate sensitivity along the fiber direction than across it, particularly at the very high strain rates.
Intermediate strain rates are perhaps more relevant to many typical impact scenarios. For instance, about half of all pedestrian impacts occur at car speeds below 25 km/h (6.9 m/s) and about 80 % occur at car speeds below 40 km/h (11.2 m/s) [41, 42]. Muscle tissue overlying the lateral and posterior surfaces of the lower limb is 25–30 mm and 50–60 mm thick respectively [43] and thus yields strain rates of 115–448 s−1. Compression tests on fresh human muscle at strain rates of 136–262 s−1 showed significant rate effects across even this narrow range of strain rates [44].
Fewer studies have examined the three dimensional properties of muscle in tension. In passive rabbit muscles lengthened quasi-statically at 0.0005 s−1, higher linear moduli and lower strains to failure were seen along the fiber direction than across it [45]. This relative stiffness was opposite to that observed in compression, where the modulus was greater across the fiber direction than along the fiber direction at similarly low strain rates [36, 37] and is consistent with a transversely isotropic incompressible material.
Much of these data are generated from ex-vivo tissue, and therefore the effect of pressurized vasculature was not included. In vivo data is generally limited to low strain rates and unidirectional loading [35, 46]. Muscle tissue properties also change considerably during rigor, however, post-rigor properties were not significantly different from pre-rigor properties at both low strain rates [47] and high strain rates [40].
21.1.5 Muscle Injury
Functional injury to muscle-tendon units occurs over a wide range of strains. Direct injury to the sarcomeres occurs from eccentric contractions; i.e. imposed lengthening during active contraction. Lengthening strains of 5–20 % in active muscle have been shown to cause injury in animal studies [48, 49]. These injuries consisted of a loss of actin and myosin interdigitation at the microscopic level. More macroscopically, damage at or near the muscle tendon junctions has been observed at about 25 % strain in passive muscle [50]. These injuries result in small focal areas of muscle fiber rupture and hemorrhage near the distal myotendinous junction. Complete rupture of passively stretched muscle also occurs at the distal myotendinous junction at strains varying from 73 % to 225 % depending on the specific muscle [51]. Increasing complexity in muscle architecture (fusiform, uni-pennate, bi-pennate and multi-pennate) was associated with increasing strains to rupture. In active muscle, the rupture force increased by about 15 %, but the strain to failure and failure site did not vary [52]. These data indicate that a muscle’s passive force rather than its active force dominates its failure [53]. All of the tests described above were performed at strain rates at or below 0.7 s−1. At higher strain rates (~6 s−1), ruptures in passive muscle have also been reported in the muscle belly [54].
Direct injury to muscle can also occur from blunt trauma. Tensed muscle distributes the impact force more broadly than relaxed muscle [55]. In simulated bumper impacts into the lower leg at 2.5 m/s using ~1.8 kg impactors, larger muscle compression occurred in relaxed muscle than in tensed muscle for posterior impacts to the gastrocnemius/soleus muscles, whereas no difference was seen between relaxed and tensed muscle in lateral impacts over the thinner peroneus longus and/or extensor digitorum longus muscles [43]. These findings suggest that muscle could have a protective effect on the underlying bone, but this protective effect varies with muscle thickness and level of activity.
21.2 Muscle’s Effect on Accidental Injury
Since peak muscle forces are less than the peak inertial or contact forces that can develop in many impact situations, we expect muscle forces, whether from pre-impact bracing or reflex activation, to have a greater effect on injury in low severity events than in high severity events. Moreover, given the large proportion of total body mass made up of muscle and the fact that muscle is not rigidly coupled to the skeleton, the amount and activation level of muscle can alter the effective mass of the body during an impact. This behavior differs from fat and other tissues whose effective mass cannot be voluntarily changed.
21.2.1 Head and Brain Injuries
Head and brain injuries generally occur as a result of head impacts. Increasing injury frequency and severity are generally related to increasing impact force and the induced kinematics. Neck muscle forces can alter the head kinematics, but the magnitude of the neck muscle forces in relation to the head mass (~3.5–4.5 kg) suggest that neck muscles will have the greatest effect at the least severe end of the injury spectrum, i.e., concussions. Moreover, since head impacts typically have durations (5–15 ms) shorter than neck muscle reflex latencies, neck muscles can play a role only if tensed before impact.
Sport-related concussion occurs more frequently in females than males [56, 57]. One potential explanation for this phenomenon is the greater neck muscle strength in males than females [58]. This hypothesis is supported by a model showing that increased neck stiffness (achieved by increased neck muscle strength and activation) can attenuate head kinematics, which could reduce the risk of concussion [59].
If strong, active neck muscles can attenuate peak head kinematics, then players with strong necks who are anticipating an impact might be expected to experience lower severity head impacts than players with weak necks who are not anticipating the impact. Static neck strength measured in flexion, extension, anterolateral flexion, posterolateral flexion and axial rotation did not correlate with peak head linear or angular acceleration in youth hockey players [60]. Anticipation, however, decreased angular head acceleration by about 250 rad/s2 in medium severity head impacts (50th to 75th percentile severity) in male youth ice hockey (14 years old), but a similar reduction was not observed in the most severe (upper quartile) head impacts [61].
Increased neck strength brought about by resistance training has also failed to reduce peak head acceleration. No changes in displacement or angular acceleration to forced flexion and extension of the head was observed in male and female soccer players after an 8 week training program that increased neck strength [62]. Other work in college-aged males showed that 7–10 % increases in neck strength from an 8-week training program has no effect on linear or angular head acceleration when tackling a standard football tackling dummy [63]. Acceleration data in both studies were obtained by double differentiating motion data acquired at 120 Hz, and therefore may not accurately reflect actual accelerations. Nonetheless, acceleration levels in both studies were low compared to sport-related head impacts that have caused concussion, and it is at these low impact levels that neck muscles would likely have the largest effect.
Based on current data, the postulated benefit of strong neck muscles on reducing the risk of concussion has not been detected. No studies were found that showed a role for neck muscles in reducing moderate or severe head/brain injuries.
21.2.2 Spine Injuries
Muscles are an important functional part of the spine. Muscles insert on or originate from every spinal level, with some deep muscles spanning only adjacent vertebrae and some superficial muscles spanning many vertebrae. Muscle activity is needed to achieve the dynamic equilibrium required for upright activities [64] and to stabilize the spine [65].
Muscle can in some cases cause spinal fractures in the absence of external forces. Vertebral fractures have been reported in up to 16 % of epileptic seizures [66], and although it remains unclear whether some fractures are due to the muscle contraction or a related fall, the circumstances of some cases indicate that spinal muscles alone can cause compression and burst fractures [67].
21.2.2.1 Cervical Spine
Muscles comprise the majority of the neck’s volume (Fig. 21.3). The superficial muscles, such as sternocleidomastoid (SCM) or trapezius, attach to the skull, shoulder girdle, and ligamentum nuchae but do not generally attach directly to the cervical vertebrae. Deeper muscles, such as splenius, semispinalis, longissimus, scalenes, and longus, attach on multiple cervical vertebrae. The deepest neck muscles, the multifidus muscles, also insert directly on the facet capsule of cervical vertebrae and may be relevant to injury of the capsular ligaments [68, 69]. Most neck muscles have complex architecture, with extensive internal tendons [13] and a high density of muscle spindles [70].
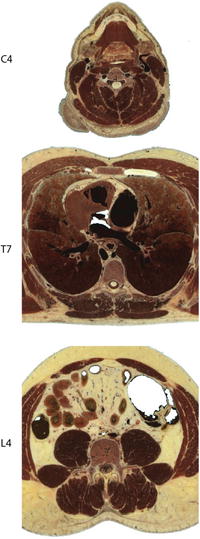
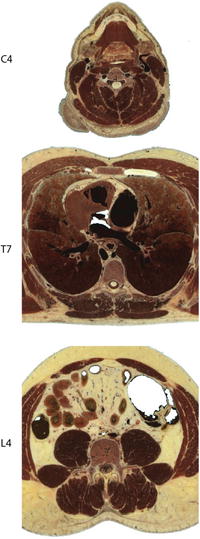
Fig. 21.3
Horizontal cross sections of the Visible Human Male at the C4, T7 and L4 vertebral levels. Note the greater muscle area surrounding the neck and lumbar spine compared to the thoracic spine
Neck muscles can potentially affect the genesis of whiplash injury in three ways. First, direct attachment of the multifidus muscles to the capsular ligament [68, 69] combined with early activation of these muscles in some subjects during a rear-end collision may increase the peak strain in the capsular ligaments [71]. Second, neck muscles are oriented primarily vertically and therefore their activation produces axial compression of the cervical spine. This increases the loads on intervertebral discs and facet joints. And third, reflex muscle activation alters the kinematic response of the head and neck during whiplash-induced motion. In subjects exposed to a series of identical perturbations without a head restraint, habituation of the muscle response amplitude by about 50 % was accompanied by a 20 % increase in neck extension and a 30 % decrease in head acceleration [72]. Whether the higher muscle activation levels are harmful or protective to other neck tissues remains unclear.
At higher impact severities, muscles also appear to affect the kinematics and the type and severity of injury. Maximum muscle activation with a reflex time of 25 ms generated better agreement between a comprehensive head/neck model and volunteer data in high severity frontal and lateral collisions [73]. Moreover, a comparison of passive and active muscles in frontal (60 km/h) and lateral (25 km/h) impacts showed that active muscles reduced the strain in many spinal ligaments [74].
Under tensile loading, maximally activated muscles in an LS-DYNA model increased the tolerance of the cervical spine from 1,800 to 4,160 N by providing an alternate load path [47]. More refined activation schemes mimicking relaxed and tensed neck muscles yielded tensile tolerances of 3,100–3,700 N [75]. In both studies, muscle activation shifted the site of injury from the lower cervical spine to the upper cervical spine, an injury site the authors reported was more consistent with those observed clinically.
Under compressive loads, simulated muscle forces also alter the injury behavior of the neck. Axial loading can buckle the spine and cause different types of vertebral fractures and dislocations depending on the line of action [76]. These injuries were produced in inverted drop tests using ligamentous spines without musculature and in a neutral lordotic posture [77]. While neck muscles cannot activate reflexively early enough to affect an injury that occurs within 20 ms of impact, the neck muscles are active and the neck posture may not be neutral following the prolonged vehicle motion leading to a diving head impact in a rollover collision [78]. Earlier inverted drop tests found that cadavers with their necks restrained by cables generating 140–200 N of pre-compression had less head rotation (unquantified) and higher head contact forces (3,000–7,100 N versus 9,800–14,700 N) [79]. More cervical spine fractures occurred in the restrained cadavers than in the unrestrained cadavers, a pattern that suggested muscle restraint could adversely affect injury potential in compressive neck injuries. Head pocketing in padding, which also constrains neck motion, has also been shown to increase cervical spine injuries [80].
In numerical simulations of diving head impacts in rollover collisions, maximally active neck muscles nearly doubled the vertebral fracture risk compared to passive neck muscles [81]. In simulations of sagittal-plane impacts from below (from 50° to 70° below horizontal), maximal neck muscle activation virtually eliminated head flexion at 5 g and reduced it by 50 % and 36 % at 13.5 g and 22 g respectively [82]. Ligament strains increased with full muscle activation during the 5 g impact, likely because the muscle induced ligament strains exceeded the impact induced strains. At the two higher impact severities, maximal muscle activation reduced peak ligament strains, likely by providing an alternate load path.
21.2.2.2 Thoracolumbar Spine
The thoracolumbar spine provides the main load path for compression and shear related to supporting the upper body. While less muscle is present in the portion of the thoracic spine that gains some of its stability from the rib cage, the thoracolumbar spine is again surrounded by considerable muscle (Fig. 21.3). Without muscle, the ligamentous lumbar spine buckles at compressive loads of 80–100 N [33], yet it supports compressive loads of 440–1,000 N during relaxed standing and about 4,000–5,500 N when lifting 10–33 kg at various speeds [83–86]. Simulating the compressive loads applied by muscles between adjacent vertebrae increased the capacity of the ligamentous spine to support a compressive load without buckling to about 1,200 N [87]. Together, these studies indicate that spinal musculature is integral to thoracolumbar spine stability, and that relatively small stabilizing forces between adjacent vertebra, possibly generated by the deep multifidus and rotatores muscles, can increase the stability and load-carrying capacity of the lumbar spine significantly [88, 89].
Cholewicki and McGill [83] showed that the lumbar spine’s stability index, which they described as the root average spine stiffness slope in all directions, increased with muscle activation, meaning that the lumbar spine became more stable with increasing external loading. This implied, somewhat paradoxically, that the lumber spine was most vulnerable to instability-induced injuries at low loads; a finding they proposed explains why some lumbar spine injuries occur during seemingly trivial tasks such as picking up a pencil from the floor.
Despite the importance of muscles in lumbar spine function and stability, there is relatively little research on the role of muscles in lumbar spine injuries. Much of the research has instead focused on occupational injuries, and in particular lifting. For lifting injuries, posture, load positioning, and expectation have been shown to affect the loads in the lumbar spine [90], and fatigue and improper muscle activation strategies have been shown to affect the risk of ligament or disc injury [83, 91].
Lumbar disc herniations, protrusions or extrusions can occur gradually or traumatically when the spine is flexed and compressed [92, 93]. Cyclical loading has generated gradual prolapses at loads varying from 2,500 to 4,500 N (Fig. 21.4) [93]. Despite testing vertebrae from all levels, these injuries only occurred at the L4/5 and L5/S1 levels. Traumatic disc herniations occurred at a compression load of 2,760–12,968 N (mean 5,448 ± 2,366 N) [92]. These injuries occurred at all five levels, though most (16 of 26) occurred at the L4/5 and L5/S1 levels (Fig. 21.4). As a result, many researchers have focused on the lumbar compressive loads.
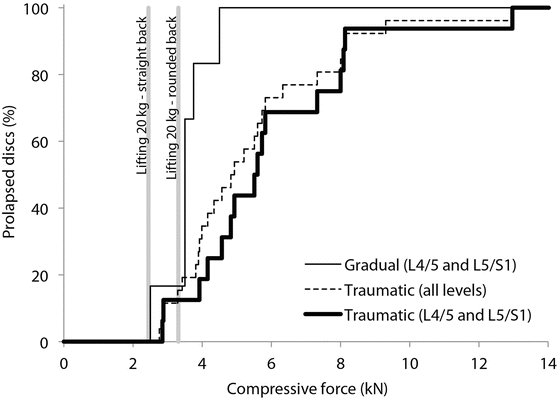
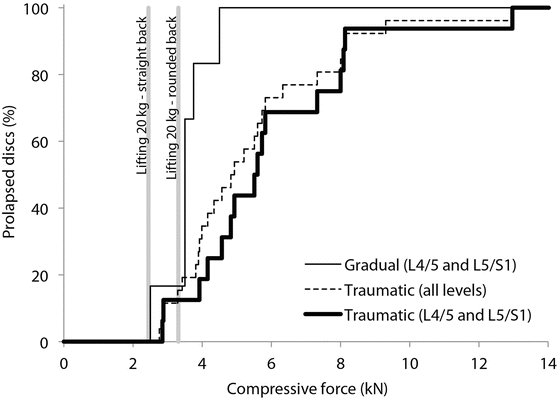
Fig. 21.4
Summary of the compressive forces generating gradual and traumatic disc herniations/prolapses at various lumbar levels [92, 93]. Also shown are the estimated compressive loads at the L4/5 disc level for lifting 20 kg with proper lifting technique (bent legs and straight back) and improper lifting technique (rounded back)
Compressive loads in the lumbar spine can be inferred from intradiscal pressure measurements. Based on stress profilometry measurements [94], the intradiscal pressure is relatively constant through the middle of the disc but falls off over the outer 3–10 mm. Assuming a linear pressure drop over the outer 5 mm of a circular disc of 22.5 mm radius [95], the effective area of the disc over which the mid-disc pressure acts is about 0.80 times its actual area. Using this effective area and intradiscal pressure data for a 70 kg male with an L4/5 disc area of 1,800 mm2, lumbar compressive loads were 144 N when lying supine, 720 N when standing relaxed, 1,584 N when holding 20 kg close to the body, 2,592 N when holding the same mass 60 cm in front of the chest, 2,448 N when lifting 20 kg properly, and 3,312 N when lifting the same mass with a rounded back [96]. Proper lifting technique involves bending the knees and lifting with a straight back. The difference between the two holding conditions and the difference between the two lifting conditions are due to different levels of back muscle contraction needed to perform each task. These muscle-induced differences are consistent with Takahashi et al. [97], who found that actual disc compression forces when standing upright and flexing forward with a rounded back were 1.43–1.74 times higher than predicted by a theoretical model without muscles. When compared to the lifting loads described above, we see that lifting improperly can generate lumbar loads in a flexed spine within the range shown to traumatically herniate some lumbar discs (Fig. 21.4).
Epidemiologically, acute lumbar disc herniations are not associated with car crashes, though they are associated occupationally with driving a vehicle [98]. Nonetheless, lumbar disc herniations are occasionally attributed to car crashes, particularly relatively minor rear-end crashes. When seated normally in an automobile, the lumbar spine is flexed, though not maximally [99]. The added flexion that arises from a slouched seated posture has not been quantified. Intradiscal pressures at L4/5 and L5/S1 in an 83 kg male were 0.5 bar (0.05 MPa) in an “ideal” automobile seat position, 0.95 bar (0.095 MPa) when the seat pan was adjusted to increase the weight borne by the thighs, and 1.5 bar (0.15 MPa) when the seat pan was adjusted to increase the weight borne by the buttocks [100]. These pressures are low compared to those measured by Wilke et al. [96], who reported 0.10 MPa lying supine, 0.33 MPa when sitting relaxed but erect in an armchair, and 0.27 MPa when “strongly” slouching in the armchair. If the pressures reported by Zenk et al. [100] are correct, they suggest a compressive load in the lumbar spine as low as 72 N (using the same assumptions as above to calculate force from pressure). Inertial lumbar spine compression in a rear-end impact is reportedly less than 870 N over a range of speed changes from 8 to 24 km/h (Gates et al. 2010) [101], although the biofidelity of the lumbar spine of Hydrid III and BioRID II dummies in low and moderate severity rear-end impact remains unproven.
During a rear-end collision, muscle activity is also present in the paralumbar muscles [102] and can compress the lumbar spine. While relaxed and seated in the vehicle, these researchers measured root mean squared (RMS) EMG levels between 1 % and 7 % (mean 2.3 ± 1.6 %) of those observed during a maximal voluntary contraction (MVC). During rear-end impacts with a speed change of 7.5–10 km/h, paralumbar EMG levels varied from 6 % to 67 % of MVC (mean 20 ± 16 %).
Estimating force from EMG amplitude is problematic, but as a first approximation, it can yield some insight into the scale of the problem. The erector spinae has a unilateral PCSA of about 11.3 cm2 (corrected for pennation angle) [103], which for a specific tension of 0.5 MPa equates to a maximum bilateral tension of 1,128 N. Assuming no contribution from other muscles, the net compressive load on the lumbar spine from the static intradiscal measurements (72 N), the dynamic dummy loads (870 N) and 20 % of the maximum erector spinae force (226 N) sums to 1,168 N. This compression level is below both the lowest compressive load causing a traumatic disc herniation (2,760 N) [92] and the lowest compressive load causing a gradual disc prolapse from cyclical loading (2,500 N) [93]. This simplified analysis suggests that low-speed rear-end collisions are not a likely cause of traumatic lumbar disc herniations, however further work is needed to better understand how an initially slouched posture in a car seat affects the static, dynamic and muscle-related loads during a rear-end collision.
The role of muscles in lumbar spine fractures is not well studied. Transverse process fractures occur in up to 29 % of patients with lumbar fractures and can be caused by either direct trauma or avulsion by excessive contraction of the psoas or quadratus lumborum muscles [104]. Beyond this relatively minor injury and the seizure-related fractures described earlier, there is little research examining the contribution of muscles to lumbar spine fractures.
21.2.3 Upper Extremity Injuries
A chief function of the upper extremity is to move and position the arm and hand. Larger shoulder muscles produce movement of the whole upper extremity while smaller wrist muscles are sufficient to move the relatively lighter hand. Major muscles of the upper extremity include: the rotator cuff muscles which provide stability and assist other shoulder muscles like the deltoid in shoulder motion; the biceps and triceps muscle groups which flex and extend the elbow; and the flexor and extensor muscle groups of the forearm that work individually or together to produce wrist flexion and extension, ulnar and radial deviation, and forearm pronation and supination. The muscles of the upper extremities are innervated by efferent nerve fibers emerging from levels C4 to T1 of the spinal cord.
Traumatic upper extremity injuries commonly include fractures, dislocations, ligament tears, and/or tendon ruptures. These injuries can occur from direct contact or indirectly through an extended or braced arm and can occur in high- and low-energy events. The upper extremity is also at risk for repetitive or overuse injures such as tendinitis, tendinosis or impingement syndromes.
21.2.3.1 Shoulder
The glenohumeral joint is the primary joint of the shoulder and has the greatest range of motion of any joint in the body [105, 106]. This range of motion comes at the cost of joint stability and injury; the glenohumeral joint is the most frequently dislocated major joint of the body [107, 108]. Glenohumeral joint stability is primarily provided by the complex interaction of static soft tissue stabilizers, like ligaments and capsular structures, and dynamic soft tissue stabilizers, like the surrounding muscles. The ligaments and capsule primarily restrain the joint at the end of the shoulder’s motion range and are lax in the mid-range of motion. In contrast, muscles provide dynamic stability throughout the range of shoulder motion but their effect is greatest in the mid-range of motion [109].
Muscles are thought to help stabilize the shoulder joint by five mechanisms: passive muscle tension; joint motion that secondarily tightens the passive ligamentous constraints; activation causing compression of the humeral head into the glenoid concavity (i.e. concavity compression); barrier effect of contracted muscles; and coordinated activation to redirect the joint force through the center of the glenoid surface [110]. Depending on joint position and the direction of injurious loading, different shoulder muscles affect joint stability to different degrees [111].
Shoulder dislocation can occur from direct trauma to the shoulder but more commonly occurs from indirect loading through the humerus [112]. In direct impact to the shoulder, the muscles and soft tissue about the shoulder absorb some of the impact energy and lessen the force applied to the shoulder. However, in lateral shoulder impacts simulating side motor vehicle collisions, the soft tissue of the cadaver shoulders (averaging 6.4 ± 4.2 mm thick) was not sufficient to prevent shoulder injuries like clavicle fracture and sternoclavicular laxity at impact speeds ranging from 13 to 25 km/h [113].
The vast majority of shoulder dislocations occur in the anterior direction from a combination of abduction, extension, and external rotation forces to the arm. These arm motions and forces indirectly load the anterior capsule and ligaments, glenoid rim, and rotator cuff [112]. Shoulder muscle activation stabilizes the joint and resists the anteriorly directed forces. The effectiveness of a shoulder muscle as a stabilizer depends on the magnitude of the muscle force and its line of action relative to the joint center [109]. Shoulder muscle forces can be broken down into joint compression and shear components. Shoulder joint compression increases joint stability by bringing the articular surfaces together whereas shear forces decrease stability by acting to displace the surfaces. For example, in a cadaveric model of anterior shoulder dislocation, passive tension of 609 ± 244 N is generated in the pectoralis major muscle which applies an anterior shear force critical to dislocation [114].
Of particular importance to shoulder stability and injury are the rotator cuff muscles: subscapularis, supraspinatus, infraspinatus, and teres minor [115]. These muscles, together with the intra-articular long head of the biceps muscle, compress the humeral head into the glenoid cavity (Fig. 21.5) [106, 110]. In addition to the cuff muscles, the outer sleeve of shoulder muscles, like the deltoid, pectoralis major and the latissimus dorsi, can also contribute to joint compression in certain shoulder positions [106, 110] although the cuff muscles are more active than these peripherally located muscles [105].
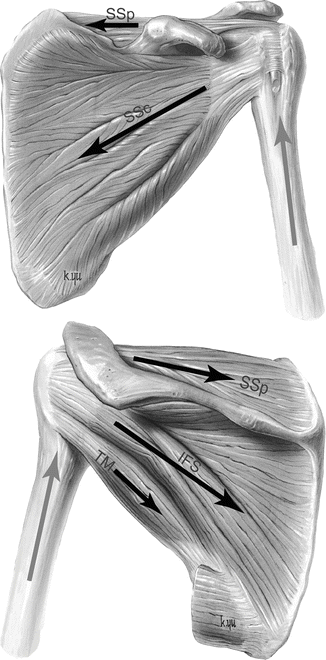
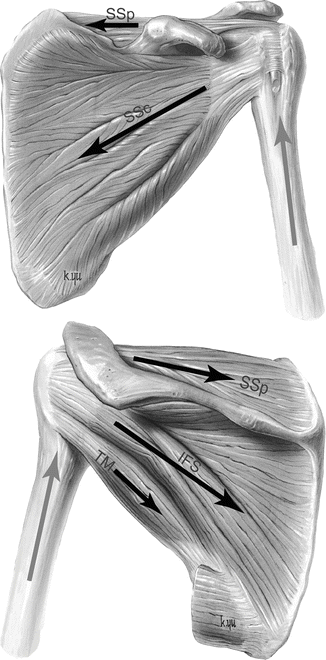
Fig. 21.5
Approximate lines of action (black arrows) of the left rotator cuff muscles compressing the humeral head into the glenoid. The deltoid muscle (not shown) can pull the humerus superiorly (gray arrows) and apply a destabilizing shear force. SSp supraspinatus, SSc subscapularis, IFS infraspinatus, TM teres minor (LifeART image copyright (2000) Wolters Kluwer Health, Inc.- Lippincott Williams & Wilkins. All rights reserved)
The rotator cuff and deltoid muscles generate about 337 ± 88 N of glenohumeral joint compression in cadaveric shoulders at 90° of abduction [116, 117] and 569 ± 141 N of compression in a forced apprehension position, i.e. forced abduction and external rotation [114]. Increased rotator cuff muscle activation increases the concavity compression in the shoulder joint and the stability of the joint to external translating forces [106, 118]. Increasing shoulder compression pre-load from 50 to 100 N increases the translational force required to cause anterior dislocation from 17 ± 6 N to 29 ± 5 N [106]. Conversely, a decrease in concavity compression through simulated inactivity of the supraspinatus and subscapularis muscles resulted in an 18 % and 17 % decrease in the force to anteriorly dislocate the shoulder, respectively [115]. In order for concavity compression to be most effective in terms of achieving stability, the joint reaction force must be kept within the glenoid fossa; this is referred to as scapulohumeral balance and depends on coordinated muscle action. This balance is affected by numerous factors including injury, instability, muscle fatigue, degeneration and altered joint mechanics.
The shoulder muscle activation level, and the resulting stability or protection of the joint, are affected by the upper extremity action being performed. In an elevated arm position, increasing handgrip force to 50 % of maximum increased rotator cuff muscle activity [119]. While the arm positions tested do not replicate a driving posture, these data suggest that gripping the steering wheel in anticipation of a crash may increase rotator cuff muscle activity and in turn increase shoulder joint stability. This has potential implications in the relative shoulder injury risk for drivers versus passengers.
To increase stability, shoulder muscles must be active before subluxation or dislocation occurs. In relaxed subjects, muscle onset times (defined as 5 % of MVC) varied from 110 to 220 ms in the anterior deltoid, pectoralis major, upper subscapuularis, biceps long head, teres minor, latissimus dorsi, lower subscapularis, infraspinatus, and supraspinatus muscles when an unexpected anterior translation force was applied to the humeral head with the shoulder in the apprehension position [105]. Anterior/inferior dislocations have been produced traumatically in-vitro in this shoulder position [120]. Although anterior muscles activated before posterior muscles, these reflex latencies are likely too long to prevent an anterior traumatic instability episode in this arm position.
Prior shoulder muscle activation shortens these latencies from 80 to 133 ms at 0 % muscle contraction (relaxed) to 64–81 ms (10–52 % faster) at 20 % of MVC and to 70–89 ms (3–41 % faster) at 50 % of MVC. Initial muscle activity increases the sensitivity of the muscle spindles, which detect the perturbation-induced muscle stretch sooner and thus provides a faster reflexive response [121]. EMD and muscle rise times increase these delays. Since the time to dislocate or sublux a shoulder is currently unknown, it is not known whether reflex activation occurs quickly enough to protect the joint.
The rotator cuff muscles are stressed and at risk for injury during many possible shoulder motions. Numerous rotator cuff injury patterns exist but usually the supraspinatus muscle is involved [122]. Rotator cuff tears typically occur in the over-40 age group due to extrinsic and intrinsic factors and are usually the end result of an ongoing process [123]. Extrinsic factors include impingement of the rotator cuff against the anteroinferior aspect of the acromion [124]. Intrinsic factors involve pathology within the tendon, usually as a result of rotator cuff overuse and overloading, and include changes in vascularity and degeneration that can lead to decreased rotator cuff function and altered shoulder mechanics [124].
In patients under 40 years old, rotator cuff lesions are more often related to activity and repetitive trauma [125]. Repetitive overhead throwing involves forceful muscular movements of the arm and shoulder and can lead to minor inflammation, subtle injury, altered mechanics and altered loading of the rotator cuff. A fall onto an outstretched arm is a common traumatic mechanism of rotator cuff injury [122], perhaps due to the shoulder experiencing the greatest deflection and absorbing the majority of the impact energy of a fall [126].
Superior glenoid labrum injuries occur at or near the tendinous insertion of the long head of the biceps onto the glenoid rim. Given the anatomic proximity and inter-connection between the labrum and the bicep tendon, the long head of the biceps muscle and tendon play a role in some labral injury mechanisms. Although the precise pathogenesis of these lesions has not yet been established, mechanisms of injury include falling or a direct blow to the shoulder, glenohumeral subluxation or dislocation, heavy lifting, and overhead racquet sports and throwing [127]. In elite-level throwing athletes, a proposed mechanism of injury is repetitive labral traction applied by the long head of the biceps tendon during the deceleration phase of throwing [128]. This is supported by EMG findings that indicate tension in the biceps and brachialis muscles is greatest at the start of the deceleration phase [129]. In a cadaveric model of lifting a large load with the arm at the side, rapidly applied traction to the long head of the biceps tendon produces SLAP (superior labrum anterior posterior) lesions at failure loads of about 550 N [130]. Introducing an inferior subluxation prior to applying biceps tension increases the incidence of SLAP lesion occurrence and is consistent with the association between labral tears and joint subluxation or dislocation [128].
Cadaveric testing has been performed to assess a traumatic labral injury mechanism in forward and backward falls onto an outstretched hand [131]. All five simulated forward falls resulted in labral injury but only two backward falls result in injury. The SLAP lesions were not created by bicep tendon tension (mean peak tension = 82.5 ± 12.1 N) but rather by shearing forces caused by impact between the humerus and the glenoid. The shearing force is affected by the rotator cuff muscles, which along with other shoulder muscles, will be highly activated in a fall. Dynamic muscle activity data during a standing height fall have not yet been published, so the effectiveness of rotator cuff muscle activity in limiting shear forces and limiting or preventing these labral lesions is not known [131].
The tensile loads applied to the bony attachments of contracting shoulder muscles can also lead to avulsion fractures, and in extreme cases, even joint dislocations [132]. For example, the superior and lateral borders as well as the inferior angle of the scapula suffer avulsion fractures at the attachment sites of the omohyoid and supraspinatus, the teres major and serratus anterior, and the teres minor muscles, respectively [133, 134]. Mechanisms for scapular avulsion fractures include: uncoordinated muscle contraction due to electroconvulsive therapy, electric shocks, or epileptic seizures; and, muscle contraction against a resisted force as a result of trauma or excessive exertion [135, 136].
21.2.3.2 Elbow
The elbow consists of the humeroulnar, humeroradial and radioulnar articulations. The radial and ulnar collateral ligament complexes of the elbow help stabilize the joint in response to varus and valgus loading. The muscles associated with the joint are the brachialis, biceps and brachioradialis muscles anteriorly; the triceps and anconeus muscles posteriorly; the supinator muscle and common extensor tendon laterally; and the common flexor tendon and flexor carpi ulnaris muscle medially [137]. The triceps and aconeus muscles extend the forearm, while the brachialis, biceps and brachioradialis muscles flex the forearm. Supination is achieved by the supinator and biceps brachii muscles while pronation is achieved by the pronator quadratus and pronator teres muscles.
Like the shoulder, the elbow achieves considerable dynamic joint stability through compression by the muscles crossing the joint, particularly the aconeus, triceps, and biceps muscles [138–141]. This is particularly true in elbow flexion where there is less bony contact [142, 143].
Elbow injury can occur from single traumatic events like a fall onto an outstretched arm, or from repetitive loading in overhead athletes like baseball, tennis, or volleyball players [144]. Common elbow injuries include tendinitis, bursitis, ligamentous strain or rupture, bony fracture and dislocation. The majority of acute elbow dislocations are posterior or posterolateral [145, 146]. Posterior elbow dislocation is often the result of a fall onto an extended and outstretched arm and hand. Even low height (6 cm) falls can generate relatively high axial compressive loads, up to 50 % body weight, at the elbow [147]. Based on the typical associated soft tissue injury patterns, axial compression, hyperextension, and valgus forces are applied at the elbow during posterior dislocation [145]. Elbow muscle activation patterns in response to this mechanism for posterior elbow dislocation have not been reported.
Although strong muscles are thought to protect the elbow, flexor muscles of the elbow provide little resistance to joint dislocation at loads up to 22 N in human subjects [148]. The limited muscle stabilization observed in this study may be partly due to specific study design factors like knowledge of loading timing, dislocation load direction, low load magnitudes and low load application rates that were tolerated by the subjects without difficulty. Therefore, the absence of effective muscle response in this study may have been due to the non-traumatic non-injurious loads applied to the elbow.
In contrast, human subjects exposed to an expected elbow extension perturbation demonstrate muscle co-contraction prior to the perturbation, suggesting muscular contribution to elbow stability may reduce the injury risk caused by sudden elbow joint loading [149]. In tests where the perturbation is unexpected, there was an increase in reflex muscle activity (defined as 25–150 ms post-perturbation onset). Better quantification of elbow muscle contribution to joint stability is currently needed to understand injury risk during sudden elbow loading [149].
Forward falls are a common source of traumatic upper extremity injury, including fractures of the distal forearm/wrist, the supracondylar region of the elbow, and the humeral neck. Peak force is highest at the wrist in experimental falls with the elbow locked in extension [126], but elbow flexion beyond 12° provides a muscle damping effect that reduces axial force to the upper extremity and delays the maximum ground reaction force [147, 150]. The effect of elbow flexion on ground reaction force at the hand varies between studies, with one study showing no effect on peak hand force [147] and others showing that fall arrest strategies like elbow flexion and reducing hand velocity can substantially reduce the peak force applied to the distal forearm during hand-to-ground impact [150, 151]. This variation may be related to the timing of elbow flexion relative to ground impact.
While elbow flexion and muscle activation mitigate injury in some upper extremity structures, it can exacerbate injury in others. Eccentric loading of the contracted triceps during a forward fall generates a tensile force at the triceps insertion onto the olecranon process [152, 153]. Pre-impact muscle activity and the stretch reflex further increase the potential for tendon/muscle rupture or even avulsion fracture, particularly in osteopenic bone [133, 154].
Muscle activation also stiffens the extremities in response to impact loading. Changes in limb stiffness may increase transmission of impact shock in the lower extremity [155], a premise that has been shown to have an injurious effect [156]. In simulated forward falls, increasing forearm muscle activation (from 12 % to 48 % MVC) stiffens the forearm and increases the rate at which the reaction force travels up the forearm [157]. These increased loading rates suggest a stiffer pathway for load transmission and an associated increase in bone injury risk [158]. Given the potentially high loading rates in motor vehicle collisions, forearm muscle activation may increase load transmission in drivers who are holding the steering wheel at impact.
Forearm fractures also commonly occur from direct contact with the steering wheel or airbag components [159], particularly over the ulna where there is little soft tissue. The typical injury mechanism for drivers is transverse loading of the forearm during the initial punch-out phase of airbag deployment. Thicker subcutaneous tissue on the underside of the forearm may attenuate the force applied by the deploying airbag reducing force transmission directly to the forearm [160]. In these tests, two cadavers with thicker subcutaneous tissue over the forearm did not sustain fractures. A similar soft tissue cushioning may also protect the humerus against injury in motor vehicle collisions [161].
Repetitive loads to the elbow can result in overuse injuries like joint laxity (from excessive ligament strain or even rupture) and tendonitis. Overhead throwing and the associated valgus extension overload can lead to elbow injury, particularly in elite pitchers. Elite pitchers generate varus elbow torques of up to 64 ± 12 Nm, which is above the 32 ± 10 Nm reported for ulnar collateral ligament rupture [162]. This suggests that muscles carry some of the load and reduce the forces on the medial passive structures of the elbow. Electromyographic studies have shown maximal activity in the flexor-pronator muscle group during the acceleration phase of throwing [163]. Simulated contraction of flexor-pronator muscles in cadavers significantly decreased elbow valgus angle and decreased medial collateral ligament strain [164, 165]. This activation may help stabilize the elbow during this motion and reduce or at least share the applied forces with the medial ulnar collateral ligament.
Excessive muscle forces or repetitive muscle contractions can also result in elbow avulsion fractures at the tendinous insertion into the bone. Although occurring infrequently overall, elbow avulsion injuries occur most commonly at the medial epicondyle in adolescents and may be acute or chronic [166]. “Little League elbow” is associated with a forceful throwing motion and recurrent or isolated contraction of the flexor-pronator muscles during the acceleration phase of throwing. These muscles attach to the medial epicondyle growth plate in adolescents and can pull the growth plate away from the bone. Fracture-separation of the medial epicondyle also occurs in adolescents during arm wrestling when one wrestler tries to force the end of the match or counter-acts a pinning move [167]. These actions represent a shift from concentric to eccentric muscle contraction, which generates peak flexor forces about 37 % greater than the forces generated by concentric contraction [168] and can change a non-injurious muscle load to an injurious one.
21.2.3.3 Wrist
The wrist consists of the distal radius and ulna, a proximal and distal row of carpal bones, and the proximal end of the metacarpal bones. The bones form a series of joints between the forearm and the hand including the radiocarpal joint (commonly referred to as the wrist joint), distal radioulnar joint (DRUJ), and the midcarpal joints [169].
Most of the muscles that move the wrist are in the forearm and originate at the elbow. The wrist extensor tendons travel over the dorsal aspect of the wrist and include: abductor pollicis longis (radial wrist abductor); extensor carpi radialis longis/extensor carpi radialis brevis (radial wrist extensors); and, extensor carpi ulnaris (ulnar wrist extensor) [169]. The main wrist flexor muscles are the flexor carpi radialis and the flexor carpi ulnaris, the most powerful wrist muscle due to its multiple short muscle fibers.
Most investigations into the stability of the wrist in response to injurious forces focus on boney geometry and interaction as well as the restraint provided by ligamentous and capsular structures. While numerous tendons cross the wrist joint, relatively few studies have addressed the potential stabilizing effect of the muscles of the wrist joint [170]. In order for relaxed muscles to contribute to the mechanical stability of the joint in traumatic situations, they must be able to sense and respond quickly enough to injurious loading conditions. Several different mechanoreceptors, which sense transient and continuous events and relay pain from excessive deformation or damage to the tissue, have been identified in palmar ligaments and suggest a protective ligamentomuscular reflex in the wrist [171].
Pre-activation of wrist muscles during a fall also influences joint stability and injury potential. In forward falls onto an outstretched hand, the palmar surface of the hand contacts the ground first and the impact force is transmitted through the scaphoid/lunate into the radius. This loading tends to rotate the scaphoid into flexion and pronation, and stretch the scapholunate ligament. Simultaneous activation of the extensor and flexor carpi muscles and the abductor pollicis longus muscle result in flexion and supination of the scaphoid [172]. Supination of the scaphoid counteracts its tendency to pronate under axial loading and maintains or moves the scaphoid to a position in which the dorsal scapholunate ligament is better protected [172].
In motor vehicle collisions, occupants aware of an impending impact brace for the collision. Bracing affects how the body interacts with the vehicle interior, the loads applied to the body and the resulting injury risk. Wrist fracture risk in side airbag deployments depends on interaction of the hand with the door handgrip and grip strength [173, 174]. In seat-mounted side airbag deployments, the airbag strikes the back of the elbow and applies an axial load through the forearm into the hand against the door handgrip. Grip strength on the handgrip affects hand, wrist and elbow kinematics and in turn the peak forearm force. In a simulation with a weak grip (10 N grip force), the upper extremity maintains contact with the airbag during deployment and the elbow is forced into full extension, which results in a high compressive load (4,760 N) to the forearm [173]. In a simulation of a strong grip (418 N grip force), the elbow slips inboard of the deploying airbag prior to full elbow extension and thus does not undergo direct airbag contact or prolonged loading. The altered forearm load path associated with the strong grip results in a peak axial forearm load about 40 % less than with the weak grip.
The scaphoid bone is the most commonly fractured carpal bone and occurs in motor vehicle collisions, sports, and in forward falls from standing height onto an extended wrist. In a fall, the soft tissue directly over the palm can play a significant role in energy absorption and affect injury risk to the scaphoid and distal radius. About 30–55 % of the total impact energy is absorbed by the skin and subcutaneous tissue, 25–40 % is absorbed by muscle and tendinous structures, and 10–15 % is absorbed by the radius [175]. As well, increased soft tissue thickness over the palmar surface, particularly between the palm and the scaphoid, directs the impact force away from the scaphoid thus potentially reducing fracture risk in forward falls [176]. As noted earlier, wrist fracture can also be affected by the muscles of the upper arm and arm position. Absorption of impact energy through a flexed elbow and muscle action can reduce the loads to the wrist and the risk of fracture from a fall compared to landing with the elbow locked in extension.
Pre-existing injury or instability of the wrist can temper the ability of muscle action to protect the wrist. Co-contraction of the wrist muscles reorients the carpal bones from their relaxed positions, however, the orientation that results from an imbalance in the pronator-supinator muscles can stretch some carpal ligaments and increase their risk for injury. In addition, contraction of the extensor carpi ulnaris (a pronator) may increase wrist instability in the presence of an injured or torn scapholunate ligament [172].
21.2.4 Lower Extremity Injuries
The lower extremities include the pelvis, hip, upper and lower leg, knee, ankle and foot. They are primarily responsible for carrying the load of the body, propelling it through space, and resisting landing forces following a jump. The lower extremities must regularly counteract ground reaction forces ranging from one to five times body weight (in some events up to ten times) while maintaining stability under the additional application of torque and other externally applied loads [177, 178]. Injuries of the lower extremities include fracture of bony structures, ligament and tendon strains and tears, and injuries to the muscles.
Active and passive muscles can mitigate lower extremity injury in several ways. In low-energy injuries, such as falls and sports-related injuries, muscle activation and recruitment can adjust posture and internally distribute loads in ways that protect from injuries. Soft tissues, composed of skin, fat, and muscle, can also act as a cushion to blunt impacts to the lower extremities but their contribution in this way is minimal. In high-energy injuries such as motor vehicle crashes, bracing by the lower extremities can protect other regions of the body by reducing peak occupant acceleration and excursion [179].
Active muscle contraction can modify the loading pattern and increase load magnitude on bony structures and ligaments of the lower extremity. Studies of long bone fractures in the leg generally do not consider the effect of active muscle contraction. However, in 3-point bending tests, thresholds for fracture are affected by axial preloading [180]. These preloads can be caused by muscle contraction [181]. During frontal motor vehicle crashes, pre-impact bracing of the lower extremity muscles often occurs [182]. Studies on cadaveric legs have shown that axial forces are amplified by simulated pre-impact muscle contraction, increasing the risk of tibia fracture [183, 184]. Compressive loading of the femur due to muscle bracing has also been used to explain femur fracture in real world frontal motor vehicle collisions where femur loads were otherwise predicted to be below injury thresholds based on external loading alone [185]. In some cases, muscle contraction can cause the traumatic injury itself. Case reports of avulsion injury at the tibial tuberosity demonstrate that muscle-generated forces alone are capable of causing traumatic injury to the lower extremities [186]. Injury risk can also be modified by acute muscle fatigue [187] and chronic differences in strength ratio between opposing muscle groups [188].
Major muscles of the hip and knee include gluteal, adductor, hamstring group, and quadriceps group muscles that control flexion/extension, abduction/adduction, and rotation of the hip and knee in addition to maintaining joint stability. Major muscles of the ankle and foot include the tibialis, peroneus, gastrocnemius, flexor, and extensor muscles that control dorsiflexion and plantarflexion of the feet in addition to other more complex stabilizing motions. The muscles of the lower extremities are innervated by efferent nerve fibers emerging from levels L2 to S3 of the spinal cord.
21.2.4.1 Pelvis and Hip
The pelvis is comprised of the two hip bones, sacrum, and coccyx. The proximal femur, which rests within the acetabulum of the hip bones, is composed of the head, neck, and trochanteric regions. These structures are the only transmission path to the ground for the weight of the head, arms, and torso. Pelvic injuries include avulsions of muscle insertions, isolated fracture of the pelvic ring, and fractures of the sacrum and coccyx. Hip injuries include traumatic hip dislocations, fractures of the acetabulum, and fractures of the neck of the femur. The greater and lesser trochanters are also susceptible to avulsion injury during vigorous athletics [189].
The pelvis and hip are held together by strong ligaments and thick surrounding muscle mass, so large forces are required to dislocate (luxate) the hip. These large forces often lead to acetabular or proximal femur fracture with hip dislocation. These injuries can occur in high-energy events such as motor vehicle crashes or low-energy events such as falls or skiing incidents. In frontal motor vehicle crashes, posterior hip dislocation often occurs through unrestrained knee impact with the dash. Other mechanisms of dislocation have been proposed where active muscle contraction is required to transmit forces into dislocating the hip. Monma and Sugita [190] proposed a mechanism of hip dislocation in frontal motor vehicle crashes requiring active bracing of the right leg against the brake prior to impact leading to traumatic posterior dislocation of the hip. Active muscle contraction forces alone, without external application of force, are capable of injuring the hip. While most acetabular fractures are due to direct impact to the hip [191], a review of seizure-induced acetabular fractures found that in some cases, seizure alone was capable of causing acetabular fracture [192].
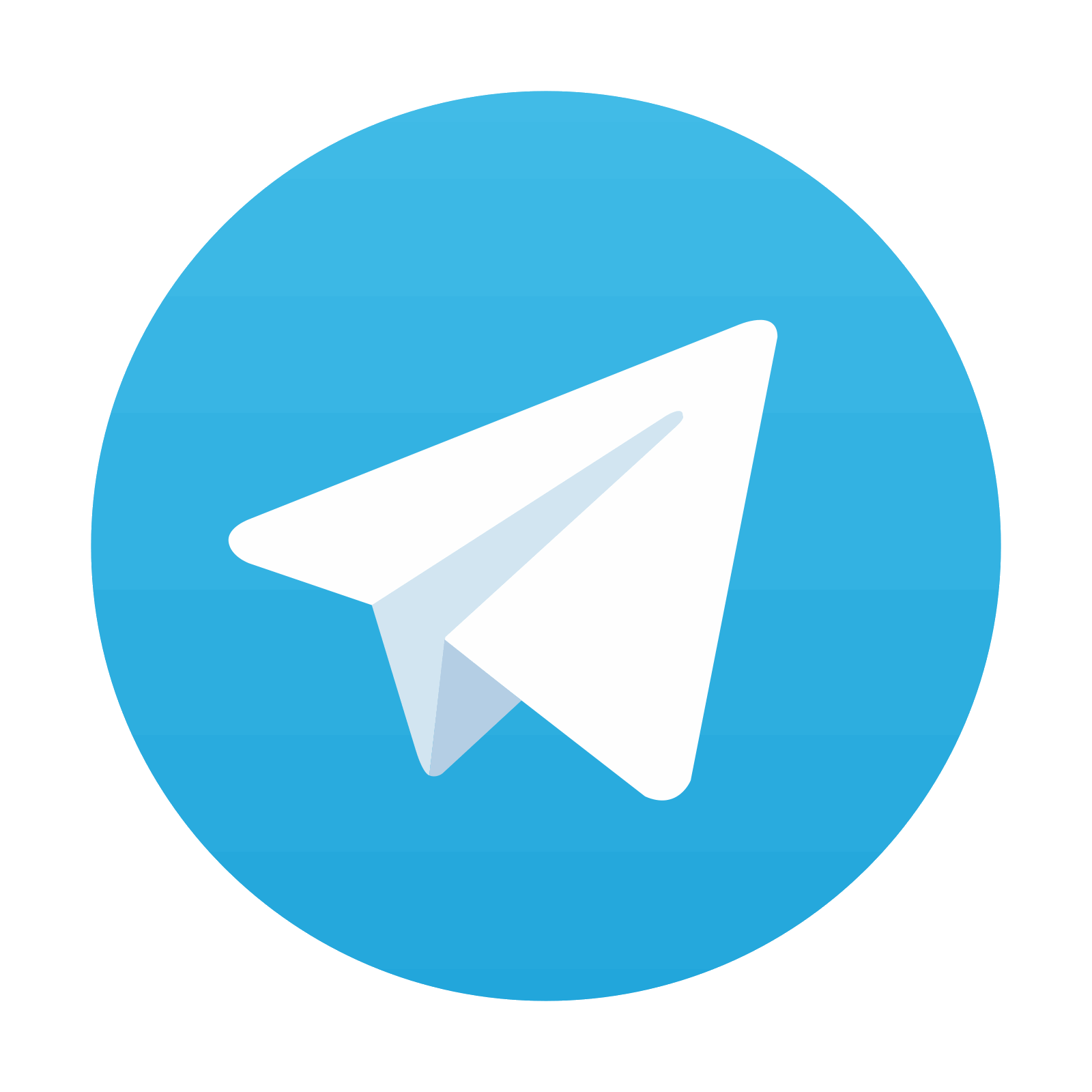
Stay updated, free articles. Join our Telegram channel
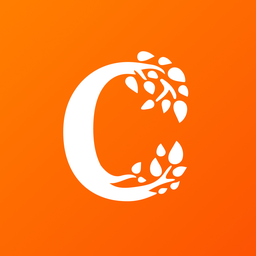
Full access? Get Clinical Tree
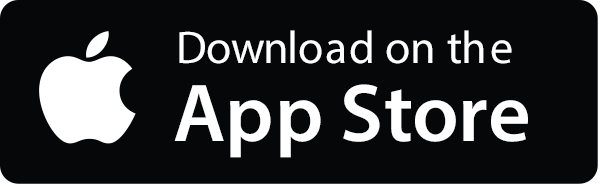
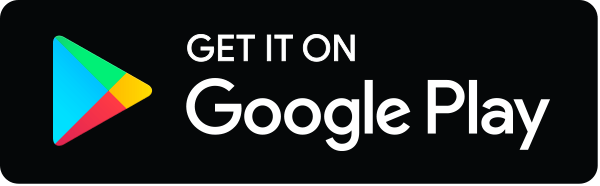