Fig. 5.1
Impact test data from Kent et al. [5] illustrating the effect of a pretensioner and load limiter. The total forward motion of the occupant relative to the vehicle is similar for the two systems, despite the significant reduction in force applied to the occupant. New restraint technology is creating the potential for even greater control and flexibility in the shape of the restraining force profile
This chapter reviews the biomechanics of restraints. The discussion includes a description of occupant kinematics for belted and unbelted occupants in frontal impacts with and without an airbag. The synergistic integration of restraint system components is a particular focus. For example, the airbag’s role in facilitating force-limiting belts is discussed. Non-frontal impacts are then discussed, followed by some special restraint design considerations, such as the occupant’s age and body habitus.
5.2 Introduction
Airbags and safety belts serve complementary functions for occupant protection since a system of technologies is needed to provide maximum safety in the wide variety of real-world crashes [17]. For example, safety belts can prevent fatal injuries in many crash modes, but they must be worn by the occupant to be effective. Conversely, airbags require no action by the occupant, but they do not provide protection in all impact scenarios. Even as many thousands of lives are being saved by belt use and supplemental airbags, however, serious injury and fatalities still occur in severe crashes. Likewise, any restraint system can have a negative effect in certain instances. Seat belts can cause injurious thoracic, abdominal and, more rarely, neck loading, particularly if they are misused. In particular, injury can result from or be exacerbated by placement of the shoulder harness under the arm and wearing of the lap-belt high on the abdomen with poor seating posture. An airbag can also cause injury if the occupant is in the path of deployment. Therefore, the airbag design requires a balance between a long fill time to reduce the risk of inflation injury and a rapid inflation to fill the space between the occupant and the interior. The design, implementation, and legislation of these restraint systems therefore require consideration of a complex web of biomechanical and other tradeoffs.
5.3 Seat Belt Biomechanics and General Restraint Maxims
The fundamental principles of crash energy management are most easily illustrated with a collinear frontal impact between a moving vehicle and a fixed rigid barrier. The kinetic energy of the vehicle must be dissipated through the process of work. Most of that work is done by the deforming structure of the vehicle. Likewise, the kinetic energy of the occupant must be dissipated through the application of forces to the occupant. In broad terms, anything that applies these forces can be considered to be part of the restraint system, including the seat, knee bolster, steering wheel, seatbelt, and airbag. The vehicle structural mechanics and the restraint mechanics are inextricably linked as the vehicle’s deceleration during an impact is dictated in large part by its structure and the vehicle deceleration can be thought of as an input that drives the restraint system’s development of forces on the occupant. These two components of the vehicle’s occupant protection system (structure and restraints) are designed to maximize the dissipation of the occupant’s kinetic energy while minimizing the risk of injury from the restraining forces.
These fundamental concepts of occupant restraint can be illustrated if the speeds of two particles are plotted as a function of time. Consider the vehicle as a particle and the occupant inside the vehicle as another. Assume that the motion is one-dimensional and that the vehicle and the occupant are both translating at a speed of 48 km/h. If the vehicle strikes, for example, a rigid fixed barrier, it will rapidly undergo a change in speed from 48 km/h to 0 km/h. For a contemporary vehicle front end, this will occur over approximately 100 ms, though this time varies depending on the nature of the vehicle’s structure. If the front end of the vehicle crushes 60 cm during this impact, the vehicle will decelerate at an average of approximately 15 g. All else equal, if it crushes less it will have a greater average deceleration, and vice-versa. The occupant’s speed over the ground will remain constant until the occupant is acted upon by a force, but the deceleration of the vehicle will build relative speed between the vehicle and the occupant and the occupant will begin to translate relative to the vehicle. The force acting on an unrestrained occupant will be virtually zero until the occupant strikes the interior of the vehicle. Assuming that the occupant is 60 cm from the interior at the start of the impact, the occupant will strike the vehicle interior after approximately 100 ms, and the relative speed between the occupant and the vehicle will have become close to the pre-impact speed of the vehicle over the ground. The occupant will then rapidly undergo a change in speed from its pre-impact speed to zero. This scenario is shown in the top plot of Fig. 5.2.
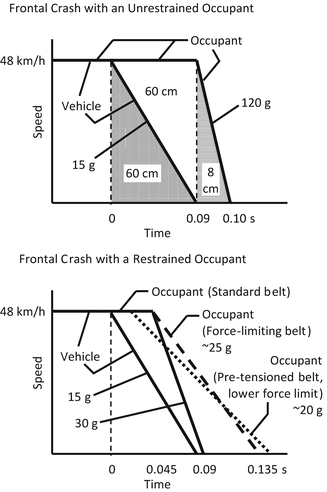
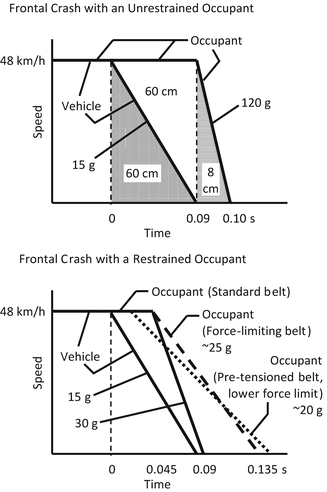
Fig. 5.2
Particle speed plotted as a function of time to illustrate the fundamental behavior of an unrestrained (top) and restrained (bottom) occupant in a frontal impact
Contrast this scenario with the case of a restrained occupant illustrated in the bottom plot of Fig. 5.2. In this case, again the vehicle will rapidly decelerate upon impact and the occupant’s speed over the ground will remain constant until the occupant is acted upon by a force. If that force is generated by a restraint system, it can occur earlier and in a more controlled manner than the forces that are generated by a strike with the vehicle interior. This will generate a more gradual change in the occupant’s speed during the impact. The restraint system will begin to generate force on the occupant a few milliseconds after impact (pre-tensioners and occupant positioning systems reduce this time) and apply that force over the entire available distance between the occupant and the vehicle interior. With a well-designed, contemporary restraint, the occupant’s deceleration during the impact may actually be less than the deceleration of the vehicle since the occupant has a greater distance available over which to decelerate (the vehicle decelerates over the distance that it crushes while the occupant decelerates over that distance plus the initial space between the occupant and the vehicle interior). The peak force acting on an unrestrained occupant can be several multiples, even orders of magnitude, greater than the peak force acting on a restrained occupant.
These fundamental concepts, combined with general considerations of human anatomy and injury mechanics, suggest several general restraint maxims:
Restraint Maxims (Mechanical)
The time over which restraint force is applied should be maximized. This minimizes the magnitude of that force and hence the associated risk of injury.
The distance traveled by the occupant over the ground as restraining forces are applied should be maximized. This minimizes the magnitude of the applied force and maximizes the work performed to dissipate the kinetic energy of the occupant. This distance is limited by the available distance between the occupant and the vehicle interior and by the distance available for vehicle crush.
The restraining force should be maximized below an injurious level and should be applied as early as possible. This maximizes the work performed to dissipate the kinetic energy of the occupant and minimizes the required distance between the occupant and the vehicle interior.
Restraint Maxims (Anatomical)
Body articulations, local deformation, rate of deformation, and acceleration of the occupant should be minimized to minimize the risk of injury.1
The restraining force should be applied over the largest possible area. Load distribution reduces body deformation and surface pressure for a given magnitude of force.
The magnitude of restraining forces applied to a body region should not exceed the force tolerance of that region. This generally results in restraining forces being applied primarily to the bony structures of the head, shoulder, upper thorax, pelvis, and femur as these are most able to bear load without injury.
A series of papers in the mid-1970s described motion sequence and restraint design criteria to focus restraining loads on biomechanically favorable anatomical locations and to minimize relative displacement between individual body parts [23–25]. These criteria have formed an important biomechanical foundation for restraint design, and remain key aspects of contemporary restraint design and evaluation [3]. The key concepts propounded in these studies were the trajectories of the hip and shoulder, which should conform to the following:
1.
Vertical downward motion of the hip should be avoided. A “gentle upward motion” is allowable if it does not exceed 40 mm.
This minimizes pelvic rotation and reduces the tendency for the lap-belt to slide off the ileum and directly load the abdomen.
2.
The torso should be pitched forward to a vertical orientation or beyond when the shoulder belt force and the forward excursion of the chest reach their peak values.
Forward rotation of the upper torso, to slightly greater than 90° upright posture, directs a major portion of the upper torso restraint into the shoulder (Fig. 5.3). The second criterion was not discussed by the original authors for the case of a primarily trapezoidal shoulder belt force-time history that has a long period of essentially constant force, such as that generated by some force-limiting belt systems. Regardless, it seems reasonable to assert that the recommended temporal relationship between torso pitch and forward excursion remains valid regardless of whether a load limiter is used.
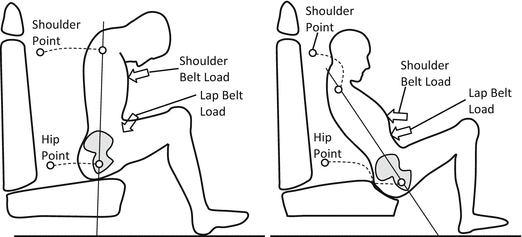
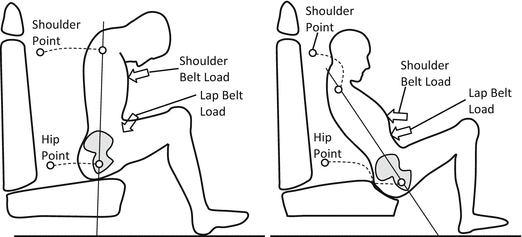
Fig. 5.3
Fundamental kinematics of “high quality” (left) and “low quality” (right) belt restraint in a frontal crash [23]
Finally, though not discussed explicitly in the Adomeit studies, it seems axiomatic that, all else being equal, a third criterion could be stated:
3.
A restraint that allows less excursion, especially of the head, is preferable.
In a frontal impact, a snug-fitting lap-shoulder belt ties the occupant to the passenger compartment and allows the occupant to “ride-down” the crash as the vehicle front-end crushes. This coupling and ride-down decelerate the occupant more gradually than is possible with energy-absorbing interiors. Even with shoulder belt restraint, however, there is forward excursion of the torso and, in particular, movement of the head and neck toward the steering wheel or windshield. Figure 5.4 shows three time-points in a frontal crash of a lap-shoulder belted rear-seat occupant and highlights the forward lean of the upper torso, the flexion of the neck, and the excursion of the head. While this kinematic is more favorable than the consequence of an unrestrained occupant striking the interior surfaces at high speed, there is still the potential for head and face contact with the vehicle interior and for inertial injuries of the neck. In fact, significant neck injuries have been generated in cadavers with restraint mechanics that fail the Adomeit criteria [26]. Figure 5.5 shows the same type of crash sequence as Fig. 5.4 with a combined driver airbag and lap-shoulder belt restraint. Early in the crash, sensors detect the severity of the crash and activate the inflation of the airbag if the collision severity is above a set threshold. This causes a rapid filling of the bag as it deploys out of the steering wheel hub. The bag then loads the head, neck, and torso to restrain the upper body in ways not possible with only the shoulder belt. Vent holes in the back of the airbag relieve pressure and absorb energy such that, even after occupant rebound, the airbag continues to deflate. This type of restraint provides a benefit to the occupant via several mechanisms, as described in the following section.
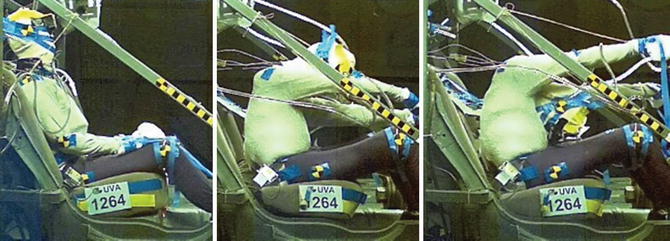
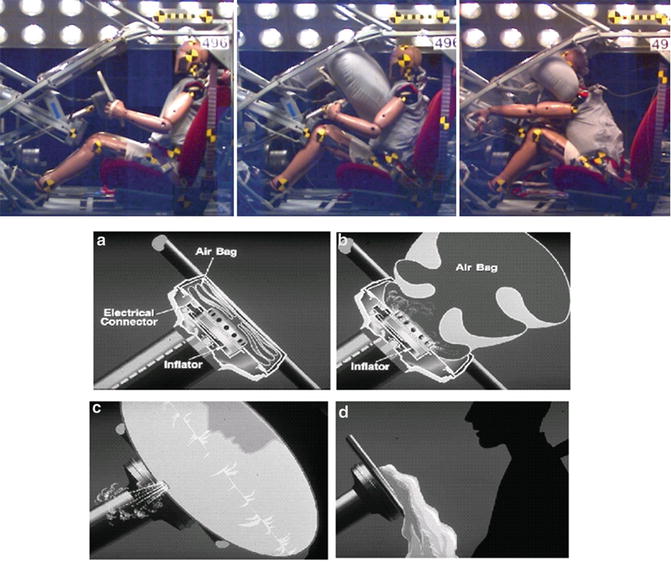
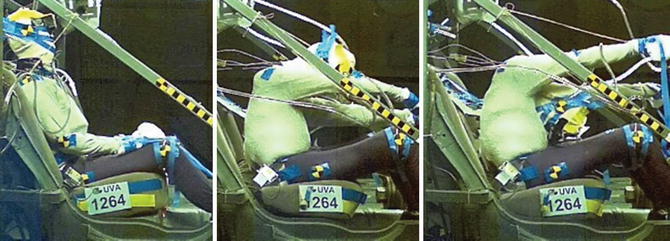
Fig. 5.4
Kinematics of a belted occupant without an airbag in a frontal collision [26]. Note the neck loading via head inertia and the potential for head/face contact with the vehicle interior
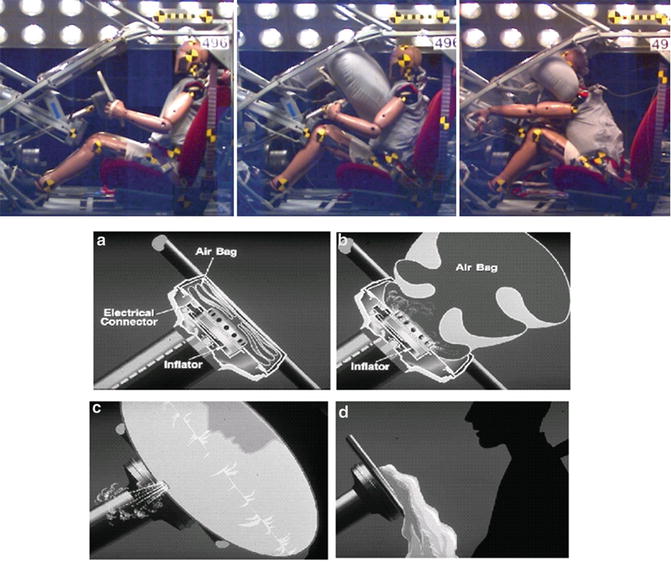
Fig. 5.5
Driver interaction with an airbag in a frontal collision. Inertial neck loading and the potential for head/face contact are mitigated and work is performed to dissipate kinetic energy. (a) Stored bag prior to impact. (b) Airbag inflation. (c) Kinetic energy dissipated via venting. (d) Airbag deflated after venting
5.4 Inflatable Restraint Biomechanics
In the early days of airbag development, belt use was low and showed no signs of increasing in the near term [27]. Low belt use rate was the original impetus behind the development of airbag systems since they overcome the primary weakness of belt systems: to be effective the occupant must fasten the belts in advance of the crash. Using a pyrotechnic device to generate nitrogen gas, a bag can be rapidly inflated during the early phase of vehicle frontal crush without action by the occupant. The bag then “fills” some of the space between the occupant and the interior, which couples the occupant to the passenger compartment and achieves the safety benefits of ride-down and load distribution. Energy dissipation is achieved via venting since a non-vented bag acts essentially as an elastic spring once it is inflated [28].
For unbelted occupants, the airbag’s principle benefit is load distribution and attenuation on the thorax. Despite low belt use being the original motivation, however, the airbag also provides a safety benefit for a belted occupant, albeit via a different mechanism. For belted occupants, the principle benefits of an airbag are due to mitigation of head contact and neck loading. The primary mechanisms by which airbags protect occupants are outlined below.
5.4.1 Head and Face Contact Mitigation
First, the airbag provides primary restraint to the head and face so that contact with the steering wheel is mitigated. This benefit has been documented thoroughly in frontal impact tests. This is not to say that an airbag precludes any risk of head or face contract, especially in the absence of concomitant belt use. Crandall et al. [29] and others have observed that head and face injuries are over-represented in unbelted occupants with an airbag compared to belted occupants with no airbag. Berg et al. [30] presented a series of seven cadaver tests, where an airbag restraint with no belt was used. They noted head impacts into the upper region of the windscreen or the roof in all tests. Depending on the size of the occupant, the severity of the collision, the geometry of the steering column and the vehicle interior, and the characteristics of the airbag, it is possible for an occupant to translate vertically over the airbag and sustain head or face loading via the steering wheel or the windshield/windshield header (Fig. 5.6). This injury mechanism emphasizes, again, the importance of the belt as a primary restraint and the airbag as a supplemental restraint. With a properly positioned belt restraint, the whole-body motion shown in Fig. 5.6 does not occur.
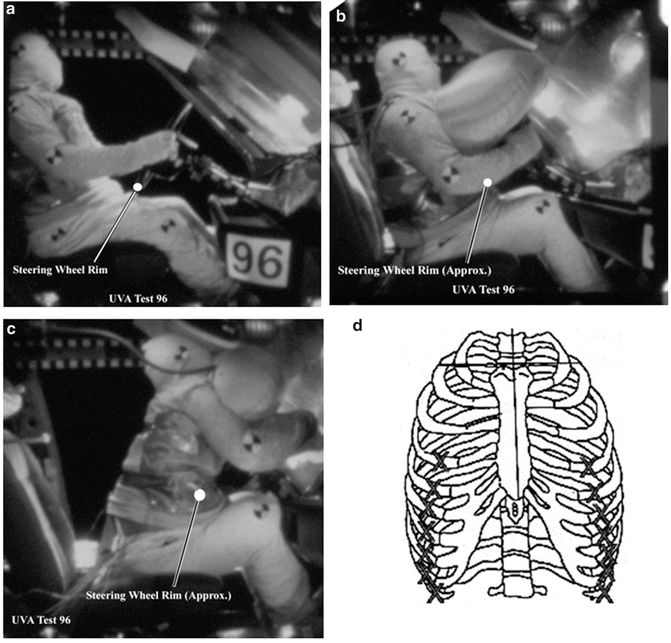
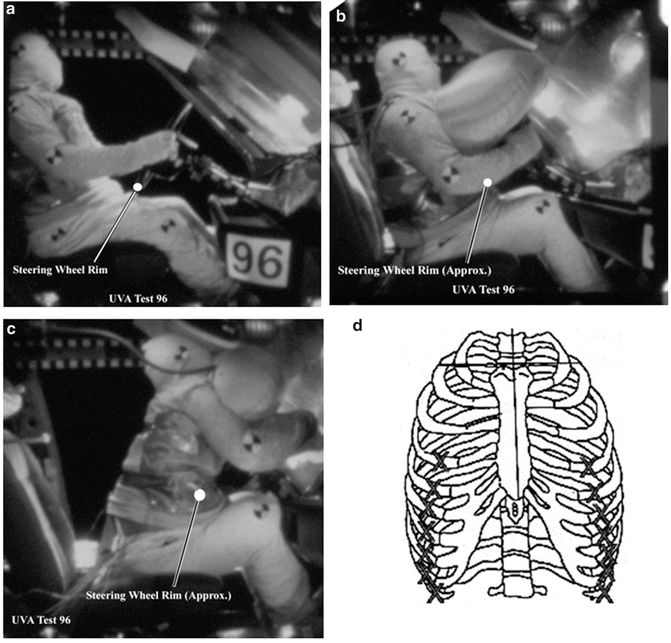
Fig. 5.6
Unbelted cadaver translating vertically over the airbag and sustaining a substantial head strike on the header (~175 g acceleration spike recorded at the first thoracic vertebra). Also note the steering wheel loading on the inferior anterior thorax, which resulted in multiple bilateral rib fractures, as shown. Adapted from Kent et al. [31]. (a) time = 0 ms. (b) time = 68 ms. (c) 122 ms. (max. chest deflection) (note head strike). (d) 14 rib fractures (7 displaced)
5.4.2 Load Sharing, Force Distribution, and Work
Second, the airbag distributes forces on the chest and shares loading with the belt system. Numerous studies have shown that cadavers restrained by a belt and an airbag sustain lower levels of injury than cadavers restrained by a belt alone or by an airbag alone [32] [7, 21, 33]. Shoulder injuries related to belt loading also seem to be reduced with the addition of an airbag restraint [34] (Fig. 5.7). These injury reductions are primarily due to the load sharing and force distributing effects of an airbag. It is well established that a person’s tolerance to a force applied on the thorax is highly dependent upon the area over which that force is applied. For example, Patrick et al. [35] performed a series of sled tests with embalmed cadavers impacting padded load cells anteriorly. They found that a 3.3 kN hub load to the sternum resulted in minor trauma, while similar injuries required approximately 8.8 kN if the load was distributed over the shoulders and chest. Bierman et al. [36] tested volunteers with a drop device that loaded a four-point belt harness 490 cm2 in area. Painful reactions and some minor injuries occurred when loads exceeded 8.9 kN. When the load area was increased to 1,006 cm2, loads up to 13.3 kN were sustained without pain or injury. The potential exists, therefore, to increase substantially the work done on an occupant and to perform this work at a non-injurious force level if the force can be distributed over the entire anterior thorax.
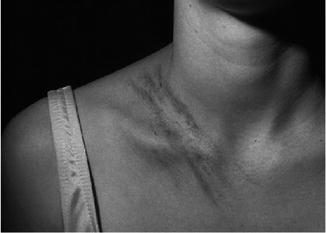
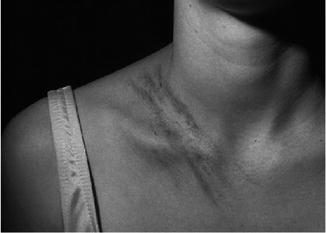
Fig. 5.7
Abrasion from shoulder belt loading. Belt injuries include fractured or dislocated clavicle, rib fractures, or others. The presence of an airbag can reduce the frequency and severity of belt-related injuries
This work can be increased even further, without increasing thoracic injury risk, if the occupant is tensed during the impact. In a series of human volunteer sled tests performed at 48 km/h, loading through the steering wheel via tensed arms and through the floorpan via tensed legs had a pronounced effect in terms of controlling occupant kinematics and minimizing restraining loads through the belt and airbag. The role of arm bracing has been studied by Horsch and Culver [37] and shows a considerable ability to restrain the upper torso away from the steering wheel when the driver is alert of the pending crash. Similar effects can be seen with bracing by volunteers in simulated crashes [38]. However, the proportion of supplemental restraint by bracing decreases with increasing crash severity, simply because the occupant’s strength becomes negligible compared to the inertial forces exerted on the upper body. In these cases, the airbag plays a greater role. Nonetheless, comparison of the tensed human volunteer in Fig. 5.8 with the atonic cadaver in Fig. 5.6 indicates the substantial effect of musculature in a crash as severe as 48 km/h. Armstrong et al. [40] estimated that as much as 55 % of an occupant’s kinetic energy in a tolerable frontal impact may be dissipated via the work done by “propriotonic” restraint.
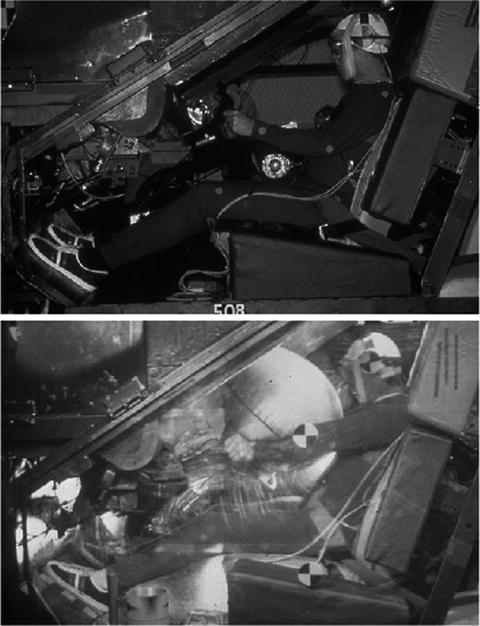
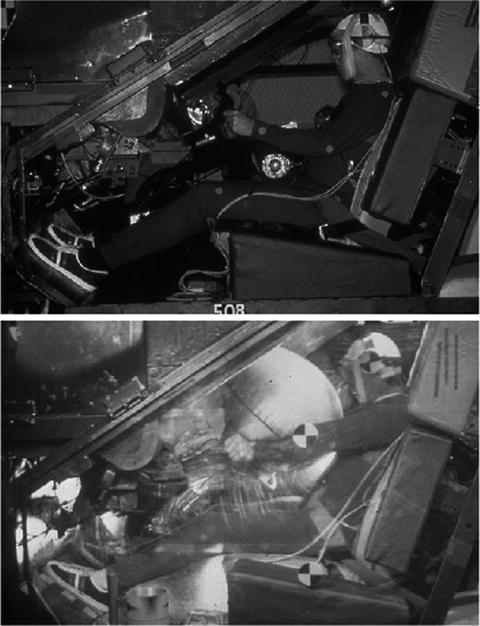
Fig. 5.8
Human volunteer frontal sled test (48 km/h) showing airbag system feasibility and pronounced effect of musculature, which reduces restraint loading relative to a dummy or cadaver test (From Holloman Air Force Base tests of the GM driver air cushion described in Smith et al. [39])
The load-sharing characteristics of airbags have led to the observation that thoracic acceleration, a widely used injury indicator, may not reflect an airbag’s benefit to the occupant. By distributing forces on the chest, the total force on the chest can be higher than the force generated by belt loading, thereby resulting in higher chest acceleration. Furthermore, the fundamental of upper torso lean is an important attribute of belt restraint and loading through the bony structures of the body. Forces concentrated on the shoulder or upper thorax are more tolerable than forces concentrated lower on the thorax or abdomen. With this background, it is necessary to evaluate chest acceleration critically when comparing belt-dominated loading with airbag-dominated loading since a higher level of force, and therefore acceleration, can be tolerated if the force is distributed or if the torso is pitched forward. Grosch [41] found that the expected benefit due to a supplemental airbag was not reflected by the acceleration levels measured in laboratory tests and concluded that it is necessary to consider other indicators of injury risk. Others have observed that the chest acceleration peak due to bag slap during deployment can be the global maximum, though the magnitude of this peak (approximately 50 g) is insufficient to cause acceleration-induced injury [42]. An acceleration peak such as this, having a different cause than a comparable sled test with belt loading, can lead to misinterpretation of the relative benefits of different restraint conditions. In the Grosch test series, chest acceleration and chest deflection exhibited contradictory trends, with acceleration increasing when an airbag was added, while deflection decreased. Further complicating the assessment is the fact that this phasing is different for drivers and passengers. Driver-side airbags typically deploy rearward nearly far enough to load the occupant prior to any forward translation of the driver in the vehicle. The result of the driver’s proximity to the deploying bag is that the airbag loading begins sooner after the bag deploys (approximately 50 ms after impact in a 48-km/h full-frontal sled test) than it does for the passenger. The driver’s chest acceleration therefore typically exhibits a single peak, which occurs during combined belt and airbag loading. A passenger-side occupant, on the other hand, sustains primarily belt loading over the first approximately 75 ms after impact, which results in peak chest acceleration due primarily to belt loading (Fig. 5.9). The later airbag loading then typically generates a second chest acceleration peak. Depending on the characteristics of the belt, airbag, vehicle interior, and occupant, a passenger’s global acceleration maxima may occur either under belt loading or under combined loading, while a normally seated driver’s maximum acceleration occurs under combined loading. This phenomenon was observed by Vezin et al. [43] and its importance for thoracic injury prediction in the laboratory is discussed in more detail by Kent et al. [31]. The combinatorial nature of belt and airbag loading also necessitates appropriate phasing of belt and airbag loading so that the combined effect does not exceed the acceleration tolerance of the human body, though cases exceeding the acceleration tolerance of a properly restrained occupant in an otherwise survivable frontal crash are assumed to be extremely rare (by inference from Melvin et al., 1998) [44]. Biomechanics researchers are currently working on the development of injury criteria that apply to combined belt and airbag systems. These criteria incorporate load distribution and load phasing within the functional form of the criterion, through multipoint measurement methods, or through the integration of the criterion within an injury risk function [5, 45].
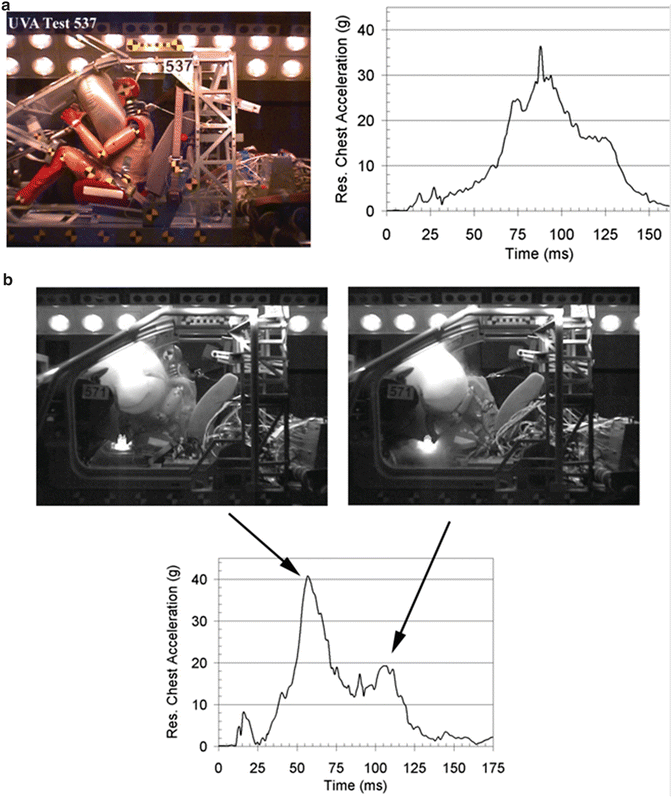
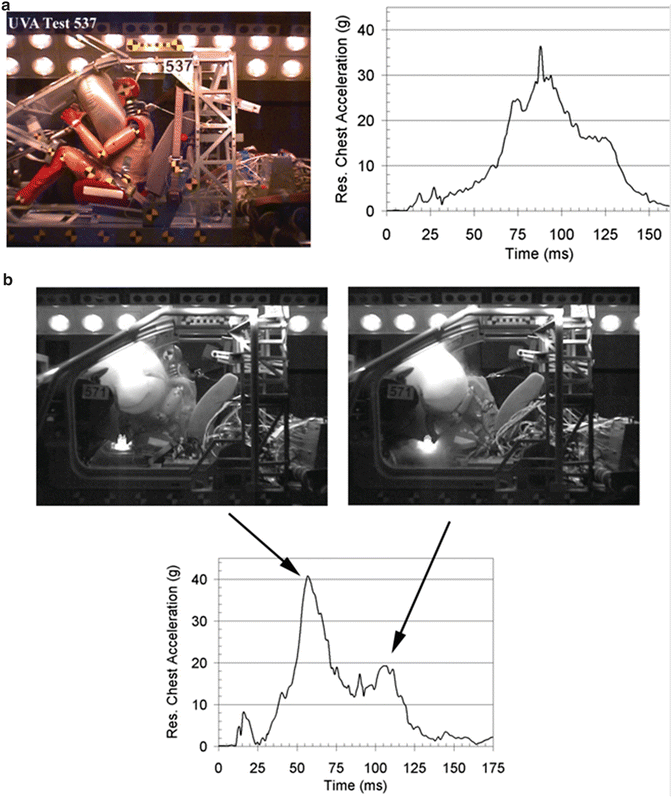
Fig. 5.9
Driver-side and passenger-side occupant kinematics and chest acceleration in a 30-mph frontal sled test with a force-limiting belt and airbag restraint system [31]. (a) Driver-side test showing Hybrid III 50th male at time of maximum chest acceleration. (b) Passenger- side showing Hybrid III 50th male at times of peaks in chest acceleration
While the airbag does share loading with the seat belt and thereby reduces the level of concentrated belt force exerted on the chest, it is typically an insufficient restraint by itself. As shown in Fig. 5.6, injurious thoracic loading can occur from steering wheel contact, even with an airbag restraint. This behavior was observed by Smith et al. [39] in volunteer tests and produced injuries in cadaver tests conducted by Yoganandan et al. [33]. Because airbags neither remain inflated nor provide lateral restraint, seat belts are needed to adequately control occupant kinematics over the range of crash types, including rollovers and side impacts.
5.4.3 Restraint System Design Flexibility
Third, an airbag facilitates the use of force-limiting belts, and allows for the use of lower force limits than would be possible without a supplemental restraint. While numerous experimental studies have documented the benefits in the laboratory, the value of lower belt force limits (e.g., 4 kN vs. 6 kN) has also been observed in the field [8]. By distributing loads, mitigating head and face contact, and sharing loading with the belt system, an airbag allows for lower levels of belt force to be applied to the occupant. This has two primary benefits. First, a force-limiting belt can be designed to yield before injurious belt forces are developed (Fig. 5.10). Second, a force-limiting belt promotes forward rotation of the torso, which generates a “higher quality” biomechanical restraint condition (Figs. 5.3 and 5.11) [23]. While a reduction of belt force is associated with a lower risk of belt-induced thoracic injury, there is a limit on how low the shoulder belt force should be reduced without compromising protection in crashes involving long-duration deceleration or multiple impacts. If the belt has yielded in a previous collision, there can be a loss of upper torso control and (with a sliding latch plate design) lap control in subsequent collisions. Since the airbag will deflate after the primary impact, it cannot be relied upon to provide restraint in secondary impacts. In this case, the belt restraint system must be available and functioning to provide retention and restraint of the occupant.
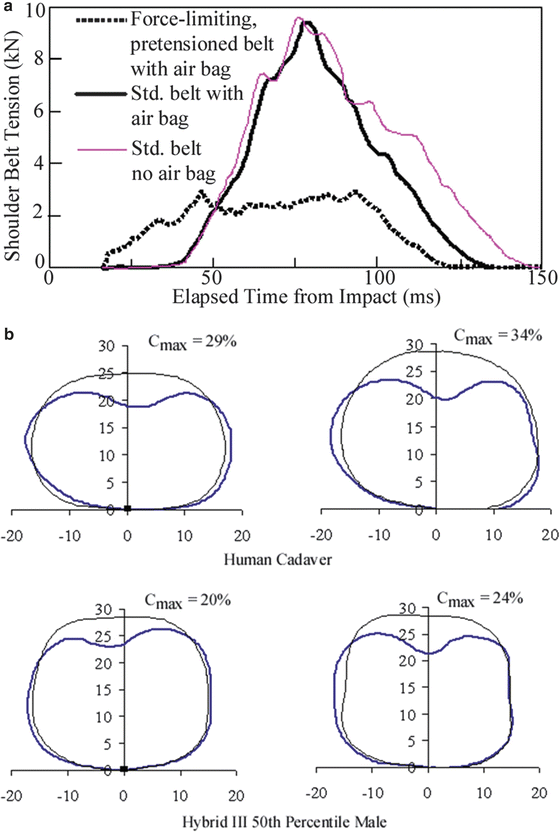
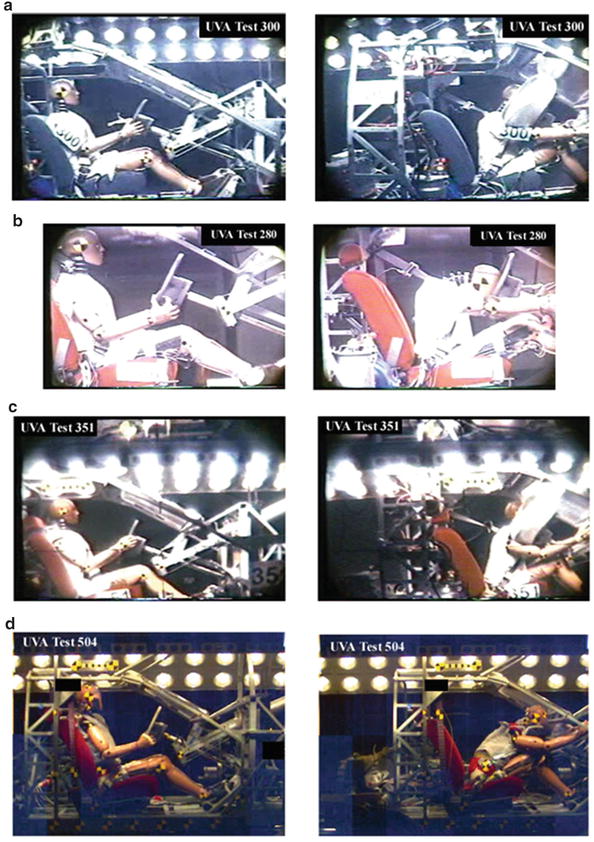
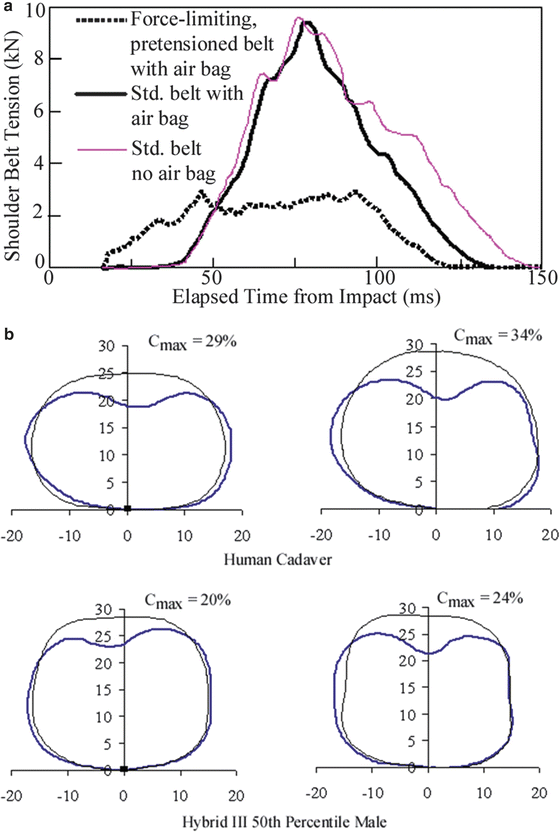
Fig. 5.10
Shoulder belt tension and thoracic cross-sectional deformation in a 48 km/h frontal impact with three different restraint conditions. (a) Shoulder belt tension. Note that the airbag reduces the magnitude and the duration of belt loading with a standard belt. Note also the substantial reduction in belt force that can be achieved with a force-limiting belt and airbag (the belt is also pretensioned in this case). (b) Thoracic profiles at maximum sternal deflection (Cmax) with 4-kN force-limiting belt (left) and standard belt (right) with airbag. Note the increased Cmax (expressed as a percent of undeformed chest depth) and decreased radius of curvature for the standard belt (units are cm)
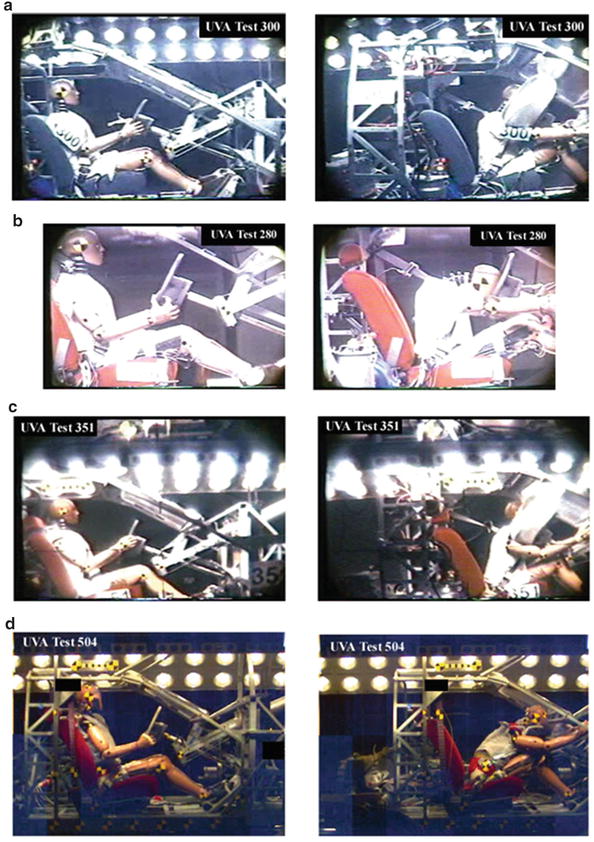
Fig. 5.11
57-km/h frontal impact restraint scenarios (50th percentile male) illustrating the benefit of an airbag. Comparing (a) and (b) shows the bag’s benefit for mitigating head/face contact and for load sharing. The inadequacy in this test of a 4-kN force-limiting belt without an airbag is shown in (d). (a) Standard belt with an airbag, at impact (left) and maximum occupant excursion (right). (b) Standard belt with no airbag, at impact (left) and maximum occupant excursion (right). (c) 4-kN force-limiting belt with an airbag, at impact (left) and maximum occupant excursion (right). Note the increased occupant excursion and torso pitch relative to (a). (d) 3.5-kN force-limiting belt with no airbag, at impact (left) and maximum occupant excursion (right). Note that this is a different dummy (THOR) than the Hybrid III used in (a), (b), and (c)
The discussion above describes the mechanisms by which airbags supplement seat belts in frontal collisions, and also illustrates how some benefit can be realized by unbelted occupants. The discussion also implies, however, the biomechanical tradeoffs associated with an inflatable restraint. First, occupant loading should occur as early as possible in a crash, so that the maximum ride-down benefit may be achieved. This emphasizes early deployment of a relatively large airbag and also the use of belt pretensioning with early activation and fast retraction to initiate early belt restraint. Second, the magnitude of restraint loading applied to the occupant should be the maximum tolerable so that injurious contact with interior components can be avoided. These requirements emphasize an airbag with relatively high internal pressure so that large forces can be developed. In contrast, the work performed on the occupant should occur over as much distance as possible prior to contact with the vehicle interior in order to minimize the magnitude of force that must be applied. Depending on the crash severity and occupant size, this emphasizes an airbag with lower internal pressures. Furthermore, occupants of different mass and geometry must be considered. It is a substantial design challenge to optimize the airbag system’s performance given the range of conditions in which it must perform, including a single crash that may have both belted and unbelted occupants.
5.5 Near-Side Collisions
Side-impact collisions can be classified based on the seating position of the occupant of interest as either near-side or far-side collisions. Near side collisions occur when the study occupant is seated on the struck side of the vehicle. Near side collisions place occupants in close proximity to the point of collision, often with less than ½ meter between the occupant and the point of external vehicle contact [46]. This limits the time and space available to provide restraint, and is reflected in the risk of severe injury or death relative to other collision types [47–49]. Approximately 35 % of car occupant fatalities occur in side-impact collisions [50]; approximately 65 % of those are near-side collisions. Injury risk is associated with impact severity and is there correlated with the presence of intrusion into the vehicle [51, 52]. Serious injuries most commonly occur in the head, chest, thorax, abdomen, and pelvis [53–63]. Fatal cases often include injury to the brain, aorta, heart, and spleen [53]. Brain injuries in near-side occupants most often resulted from striking either the pillar, the vehicle interior, or the striking vehicle (e.g., through the window) [53, 63].
The last decade has seen the widespread adoption of airbags for near-side occupant protection. Among other considerations, side airbags seek to fill the space between the body and the struck-side vehicle interior, distributing load and reducing the risk of directly impacting striking objects or the vehicle interior. These airbags take several different forms, both in shape and in the targeted body structure. Most systems can be classified as targeting either the torso, the head, or a combination of both [50, 64, 65]. Some combination systems can consist of a single airbag covering both the torso and the head. More commonly (at least in newer model years) combination systems consist of separate torso and head bags [66]. Torso airbags are mounted either in the seat back or the door [67], and vary widely in shape to engage the shoulder, lower chest, or to extend superiorly towards the head. Head protection airbags consist of either an inflatable tubular structure or an inflating curtain which extends from the roof rail down to the bottom of the window [50, 66].
Side airbags are generally protective against fatality and serious injury. Torso-only airbag systems are approximately 11–26 % effective at reducing fatalities in near-side collisions (compared to no airbag) [50, 68, 69]. Systems that include head protection are approximately 24–37 % effective at reducing fatalities [50, 69]. Kahane [50] concluded that adopting a combination of improved side structures and padding, a torso airbag, and a head curtain airbag, the fatality risk of drivers and right front passengers in all side-impact collisions (including far-side) could be reduced by 30 % for four-door cars and 42 % for two-door cars. Although data on non-fatal injuries are limited, McGwin et al. [70] suggested that side airbag availability is approximately 75 % effective in reducing the risk of any (AIS1+) head injury, and 68 % effective in reducing the risk of any thorax injury. As with other protective systems, however, the effectiveness of side airbags is closely related to the impact severity – decreasing at high impact velocities [46].
5.6 Far-Side Collisions
In far-side collisions (side-impact collisions where the occupant is opposite the struck side of the vehicle) occupant restraint occurs primarily from the seatbelt with some interaction with the seat cushion, seat back, and center armrest or console if present. The geometry of the shoulder belt – with outboard mounting of the upper shoulder belt anchor and the belt passing over the outboard shoulder – creates the potential for the belt to slip off of the shoulder [71]. This, in turn, creates the potential for greater lateral motion of the head and thorax, and greater loading of the upper abdomen and lower thorax by the lower portion of the shoulder belt. Head and thorax injuries, in general, account for over one half of all serious injuries in far-side impacts [72, 73]. Also of concern are abdominal injuries, especially to the liver and spleen [49, 74]. Much of the injury harm in far-side collisions comes from the head or thorax striking the door or window of the struck-side of the vehicle [71, 72, 75, 76], often in concert with intrusion [75]. Belt slip off of the shoulder has been attributed as a contributing factor in head injuries [77], strikes of the head and thorax against the intruding struck-side interior [72], increased loading of the lower chest and abdomen by the shoulder belt, and potential impact interaction between the struck-side and far-side vehicle occupants. Belt-slip off of the shoulder may also contribute to increased occupant excursion in oblique-far-side collisions [78].
Several studies have suggested a strong sensitivity between belt geometry and the presence of pretensioning on the risk of belt slip off of the shoulder in far-side collisions. Douglas et al. [79] presented a sensitivity study using volunteers, a Hybrid III dummy, and a MADYMO human model validated under far-side impact. That study found that both moving the D-ring rearward and adding a pretensioner tended to decrease the risk of belt slip off of the shoulder. Recent cadaveric tests have produced similar results, indicating a sensitivity to both D-ring position and pretensioning in both low-acceleration (6.6 g vehicle acceleration) and higher-acceleration (14 g) environments [80]. That study showed a decrease in lateral head excursion when belt slip off of the shoulder was prevented through pretensioning, an improved D-ring position, or both (e.g., Fig. 5.12). That study also found that even when the belt did slip off of the shoulder, the addition of a pretensioner still tended to decrease lateral excursion of the head.
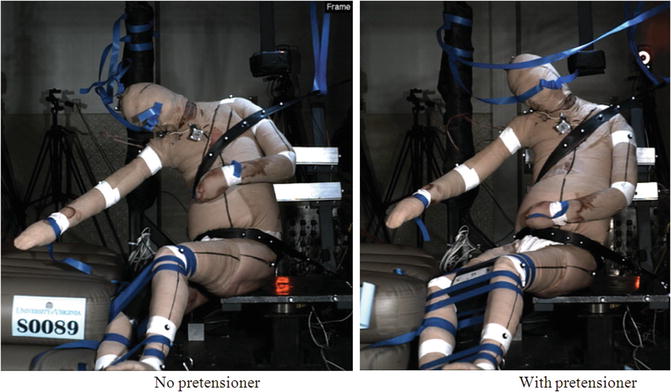
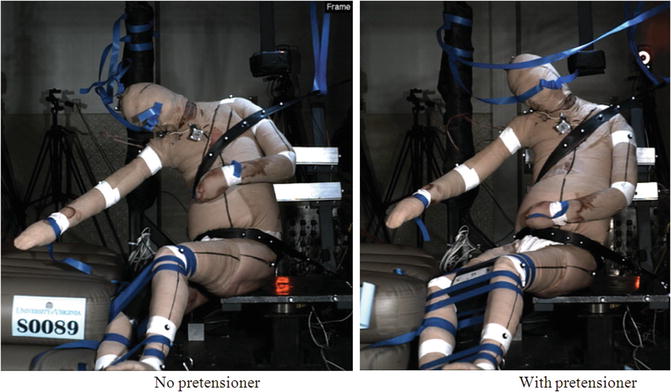
Fig. 5.12
Video captures at the time of maximum lateral head excursion in 15 km/h sled tests investigating shoulder-belt retension in a far-side collision. Left: Standard (not pre-tensioned) three-point belt. Right: same cadaver, belt with a (nominal) 1.8 kN retractor pretensioner. Notice the reduction in shoulder belt slip and lateral head motion with the pretensioning belt [80]
Other restraint concepts have been explored to prevent belt rollout (slip off of the shoulder) and reduce lateral head excursion in far-side collisions. Kallieris and Schmidt [81] performed a series of full-scale, far-side crash tests (50 km/h impacting speed; 60° and 90° impacting direction) with cadavers restrained by a reversed three-point belt system (i.e., the belt passed over the inboard shoulder). The authors concluded that the reversed belt system reduced the lateral excursion of the torso and head, however most of their cadaver exhibited AIS 1 injuries to the neck. Bostrom and Haland [2] explored the concept of adding a supplemental second shoulder belt (passing over the inboard shoulder and anchored to the seatback) to an existing three-point belt system. Bostrom et al. [72] also investigated the potential efficacy of incorporating an inboard airbag to support the torso (a “side support airbag” or “SSA”). That study suggested that the SSA may reduce AIS 3+ injuries in far-side collisions by as much as 57 %. Pintar et al. [82, 83] explored various combinations of generic side-support countermeasures in experiments with ATDs and cadavers, also suggesting that inboard torso and shoulder support may reduce lateral head excursion. Similarly, since a large portion of far-side injuries result from striking the struck-side door or window, some studies have suggested a potential benefit for far-side occupants from side-curtain airbags deployed on the struck-side of the vehicle [72]. Kahane [50] estimated a potential 24 % fatality reduction for far-side occupants when torso + head curtain side impact airbags are present on the struck side of the vehicle.
5.7 Rollover
Rollovers account for fewer than 10 % of all tow-away collisions, but are responsible for 20–33 % of fatal crashes and 15–25 % of crashes with serious injury [84–87]. Moderate-to-severe injuries in rollovers most often occur in the head, spine (including neck), and thorax [88–91]. There are several potential reasons for this disparity in risk compared to other collision modes. Some studies have suggested that rollovers tend to occur at greater vehicle speeds than normally observed in the entire population of tow-away collisions [86, 92]. Rollovers also carry a risk of ejection or partial ejection from the vehicle, especially for unbelted occupants [93]. In 2000, approximately 50 % of cases of fatally injured, unbelted occupants in rollovers in the U.S. were completely ejected from the vehicle [85]. Less than 10 % of the cases of fatal injuries to belted occupants involved complete ejection from the vehicle. Even when ejection is prevented, however, rollover collisions represent a chaotic environment containing multiple potential sources of injury. Head strikes against the vehicle roof, the side interior are common sources of head and neck injury for belted occupants in rollovers [86, 91]. Head injuries in belted occupants are also occasionally attributed to strikes against exterior structures through partial ejection [86]. Three-point seatbelts, when used, are approximately 64.2–77.9 % effective at preventing serious injury and 43.8 (far-side occupants) to 82.5 % (near-side occupants) effective at preventing fatality in rollover collisions [94].
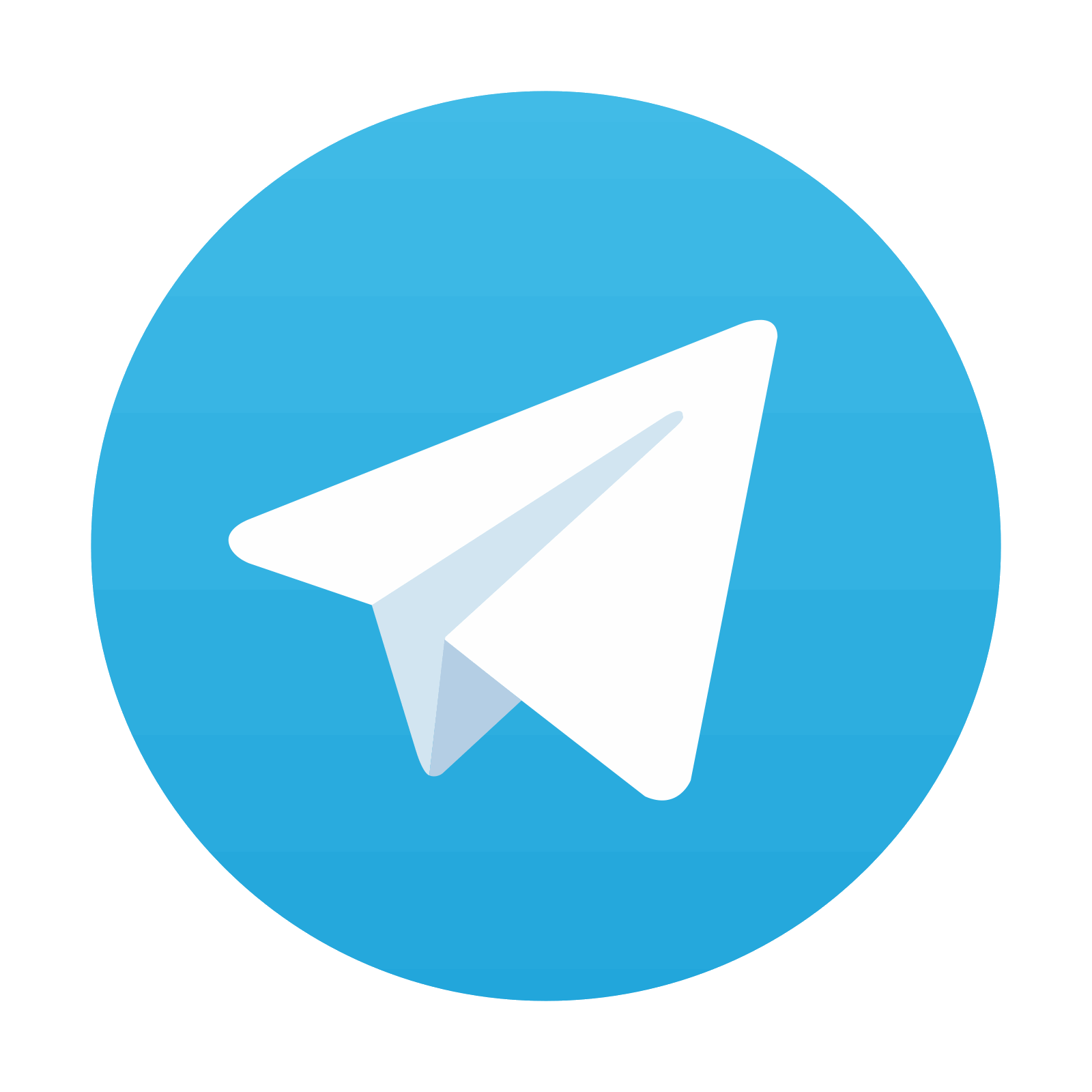
Stay updated, free articles. Join our Telegram channel
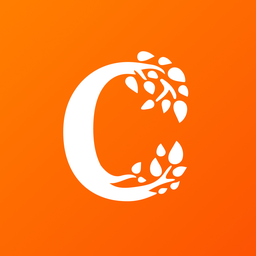
Full access? Get Clinical Tree
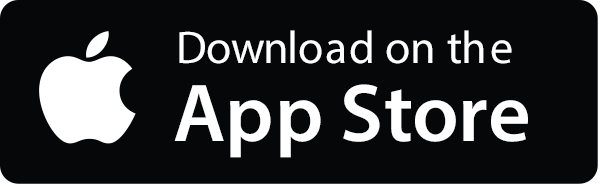
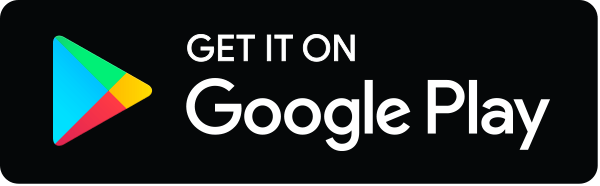