Abstract
Regenerative engineering seeks to address the most complex and relevant questions in the realm of future of regeneration, namely, how to regenerate complex structures comprised of multiple types of tissues including organized structures to improve the quality of life and the scope of structural and biological ailments that can be treated by modern biomedical science. This challenge requires a paradigm shift that incorporates a transdisciplinary approach. To reproducibly regenerate complex tissue and organ systems, a regenerative engineer must use tools from many traditional fields of study including advanced materials science, stem cell science, physics, and developmental biology, as well as observations and applications from medicine and surgery. The regenerative capability of the adult human body is surprisingly very high, and modern regenerative engineering can harness this potential to slow, halt, or even reverse the effects of injury, infection, cancer, and age-related degradation. This chapter highlights the scope of work in the field of regenerative engineering, including the development of novel materials, recent scientific breakthroughs, future research directions, as well as the potential impact regenerative engineering can have on the practice of orthopedic surgery and the ability for the orthopedic surgeon to innovate in the future.
Keywords
Bioengineering, Material sciences, Orthopedic surgery, Regenerative engineering, Stem cell, Tissue engineering
Disclosure Statement
Dr. Laurencin is an owner of hot-bone, healing orthopedic technologies, natural polymer devices, and soft tissue regeneration. He receives royalties from Globus Inc. Dr Badon has no Disclosures.
The field of regenerative engineering seeks to address the most complex and relevant questions in the realm of future of regeneration, namely, how to regenerate complex structures comprised of multiple types of tissues to improve the quality of life and the scope of structural and biological ailments that can be treated by modern biomedical science. To accomplish this task, the regenerative engineer must use tools from many traditional fields of study including tissue engineering, materials science, bioengineering, and developmental biology, as well as observations and applications from medicine and surgery. Regenerative engineering focuses on a number of areas, but the development of novel materials, in addition to redeveloping existing materials, which are then used to facilitate biological processes and the growth and development of tissues directly into functional and complete structures that are identical to their native tissues has taken center stage.
This chapter will provide some background into both the materials that have been developed as scaffolds for facilitating tissue growth as wells as insights into the biological processes that must be harnessed or modified for the cells within those regenerated tissues to reenter the cell cycle and redifferentiate into functional adult cells. In addition, we will describe the importance of innovation in this field and how regenerative engineering groups bring together scientists, engineers, and clinicians to facilitate not only sustaining or incremental improvements but also innovations that can be truly disruptive and change the way health care is delivered in the field orthopedic surgery.
Although applications should not dictate the scope or direction of basic science, it is important to highlight some of the most challenging conditions that face the modern orthopedic surgeon. This includes bone and tissue loss due to trauma, infection, cancer, and inflammation. Surgeons are skilled at rearranging and reconstructing tissues to create functional structures and are at the same time limited by the tissues present at the time of surgery. Herein lie the potential contributions of the field of regenerative engineering, to create the building blocks with which the orthopedic surgeon can reconstruct and recreate a functional musculoskeletal system, where one was missing or has been lost.
Tissue Regeneration and the Role of Regenerative Engineering
Regeneration mimics the natural processes of tissue formation that occurs during several stages of an organism’s development. Tissues normally form during fetal phase of life termed embryonic tissue formation, tissue growth and development (fetal and postnatal), remodeling (degradation-formation), and healing (repair vs. regeneration). It is important to distinguish the difference in functionality that exists in repaired tissue that forms during the normal healing process, and that tissue would be created through regenerative engineering methods.
One aspect of regenerative engineering focuses on using cues from earlier tissue-formation periods and applies them to situations and injuries that occur later. This is performed in the hopes of directing adult tissue away from simple repair, where a defect in the tissue fills with relatively biologically inactive, nonfunctional tissues such as scar. Wound healing is a conserved evolutionary process among species and encompasses processes including inflammation, blood clotting, and cellular proliferation and extracellular matrix (ECM) remodeling which leads to distinct scar formation. Regeneration on the other hand would result in tissues where the defect or injury fills with a regenerate that is indistinguishable from the original surrounding native tissues.
Tissue gaps or large scars present biological challenges that have significant clinical consequences. For example, infarcts secondary to pulmonary emboli or myocardial infarctions result in relatively noncompliant scars taking the place of biologically active tissues that reduce the overall function of the organ as the scar cannot carry out the roll of the organ. The inferior scar tissue fails to emulate the tissue’s original structure and composition. In addition to the scar replacing the native, functional tissue, the scar also impedes the function of the rest of the organ due to physical forces restricting the dynamic movement of the tissue. These gaps or scars can be focused in a single area as in the case of infarction but can also be diffusely distributed in the native, normal tissue as in the case of infection. Even focal injuries can result in congestive heart failure in the case of myocardial infarction and restrictive lunch disease in the case of pulmonary embolism. Disease processes can also result in more diffuse changes in the affected tissue. Classically in the case of hand cellulitis, the compliance of the soft tissues is reduced after the infection has cleared, resulting in joint capsule contracture as well as generation restriction throughout the skin and muscle resulting in reduced range of motion, discomfort, and difficulty with fine motor movements. Similarly, diffuse changes that affect the compliance of tissues can be seen in certain autoimmune disorders such as systemic sclerosis, a chronic multisystem autoimmune disease characterized by a vasculopathy, diffuse fibrosis of skin and various internal organs, and immune abnormalities.
Regenerative engineering seeks to create physical and biological solutions for biological deficiencies. Ideally, when materials are used, engineered matrices are involved so that they can create appropriate and appropriately timed biological and physical signals to pluripotent cells within the surrounding tissues to stimulate the formation of bioidentical tissues that are contiguous with the surrounding biological environment. The implants simultaneously act as templates and guides for tissue ingrowth and incorporation. The choice of materials used for these scaffolds, any biologics that are incorporated into their physical arrangement and structure, and the nature in which they are assembled can have dramatic impacts on the ability of the stem cells to differentiate into tissues and ultimately function in the context of the musculoskeletal system.
When a regenerative engineering problem is approached, the engineer must decide on what strategy they would like to use for multitissue structure regeneration. In nature, most tissue development occurs during embryonic development, remodeling of existing tissues, or injury healing. Each regeneration strategy may include completely differing signaling cues as to stimulate growth. For example, the engineer needs to recapitulate embryonic/developmental conditions with appropriate precursor cells and/or extracellular matrices or, if appropriate, instead provide conditions (cells and matrix) that favor tissue formation of regenerated tissue from surrounding adult tissue.
The most common approach is to establish the growth of tissues within scaffolds in vitro. This traditional approach is an important testing ground to establish proof of concept experiments that determine which developmental biochemical cues induce the desired tissue proliferation. The developmental signals used during in vitro tissue engineering and propagation can include signal transduction molecules, cofactors, and growth factors. The in vitro approach can be controlled but is limited and does not fully recapitulate the in vivo environment.
The in vivo environment may have many other, complex, structural, physical, or systemic cues that contribute to tissue development and function. For example, one must consider gradient signals associated with tissues, such as hypoxia to stimulate angiogenesis, interactions with the endocrine system or hormones, or physical load to stimulate bone growth and remodeling. However, there are other challenges associated with the in vivo environment which make experiments in live animals challenging to control and interpret.
To take advantage of the in vivo setting and thus the biological environment surrounding the injured area and mechanical stimuli, the regenerative engineer will lose some of the overall control of the experimental settings. An in vivo approach, in combination with implants that use advanced technology in materials engineering, signal transduction, and surgical techniques to facilitate the growth of complex tissues and structures, increases the scope of medical problems that can be addressed and also minimizes the duration of time tissue regeneration takes by simultaneously growing multiple types of tissue rather than needing sequential reconstructive procedures. Through the formation of implants using advanced biomaterials and techniques, the regenerative engineer is setting the stage for tissue growth and development to happen in vivo rather than solely in a petri dish.
The Clinical Relevance and Importance of Regenerative Engineering
The evolution of regenerative engineering can be marked by similar breakthroughs in biomedical history. The field of regenerative engineering can trace its origins to the 1970s and 1980s when IntegraTM (Integra Life Sciences Corporation Plainsboro, New Jersey), a collagen-glycosaminoglycan (GAG) matrix that is used to help complex wounds and burns heal, first emerged. Similar to bone, skin is another tissue that is capable of and can be steered toward regeneration rather than healing under certain conditions. IntegraTM is manufactured in the form of a wound dressing that is placed over a debrided wound. The scaffold, made up of collagen-GAG provides the needed structure that angiogenesis and ingrowth of dermal skin cells need to remodel the damaged site. Reliably, over the course of 14–21 days after placement of this dressing, fibroblasts and mesenchymal stem cells migrate into the scaffold. After migration of the precursor cells, the collagen scaffold is resorbed and replaced with the patient’s own tissue, and the wound gradually healed. This breakthrough resulted in a user friendly product that was rapidly integrated into clinical practice and is still routinely used today.
Scaffolds have found other uses in medicine and surgery including aiding in bone regeneration. Bone, when fractured, crushed, or otherwise damaged, is unique in its ability to heal without the formation of scar tissue. The stages of fracture healing includes coordinated responses of the bone marrow, bone cortex, periosteum, the surrounding soft tissues, the immune system, including regulation of cellular proliferation, and migration and differentiation of mesenchymal stem cells. However, because bone has the innate ability to regenerate, the primary use of the scaffolds was to overcome physical gaps that precluded normal healing. This is different from the goal of the development of new tissues that do not possess some regeneration capacity on their own. Examples of other tissues that spontaneously regenerate include bone, epithelia (including stomach lining, intestinal lining, and skin) and smooth muscle. Areas of active research for the development of scaffold for regeneration of tissues that do not spontaneously regenerate include articular cartilage, ligaments (predominantly collagen type I), both the nucleus pulposus, and annulus fibrosis of intervertebral discs of the spine, cardiac muscle, skeletal muscle, and nerves.
Bone is an interesting example as there are several options for scaffolds to aid in bone-defect healing. Bone is a highly structured and dynamic tissue made up of both organic and inorganic matrices. Primarily made of collage type I and hydroxyapatite (HA), it is also both innervated and vascularized as it regenerates. However, factors such as the size of defects (defects or gaps greater than 2 mm are at risk of not healing), unfavorable environments such as the collapse of tissues into defects preventing opposing ends of the bone from opposing in the case of fractures or occupying space in the case of periodontal defects, and inappropriate physical forces across the injured area in the case of unstable or over-stabilized fracture resulting in hypertrophic or atrophic nonunions (ideal strain is thought to be 2% for bone healing to occur). Options for scaffolds for bone defects include autograft, allograft, and bone substitute or synthetic materials. The tissue regeneration capacity of these bone grafts is measured in terms of their osteogenic, osteoconductive, and osteoinductive potentials. As described in a review by Liliana Polo–Corrales et al. in the Journal of Nanoscience and Nanotechnology, the table below demonstrates that many materials can be made into scaffolds conducive to bone regeneration.
Category | Material |
---|---|
Natural polymer | Collagen hydrogel |
Chitosan | |
Silk fibroin | |
Alginate | |
Hyaluronic acid | |
Peptide hydrogels | |
Synthetic polymers | Polyesters (polyglycolic acid, polylactic acid, and polycaprolactone) |
Poly(diisopropyl fumarate) (PDIPF) | |
Poly(L-lactide-co-ε-caprolactone (poly(LLA-co-CL)) and poly( L -lactide-co-1,5-dioxepan-2-one), (poly(LLA-co-DXO)) | |
Ceramic scaffolds | Bioglass |
Calcium phosphate | |
Coral | |
Metal scaffolds | Titanium/titanium oxide |
Aluminate/melatonin | |
Composites | Polymer/ceramics |
Metal/ceramic | |
Metal/polymer |
Collagen type I is also the predominant type of collagen in ligaments. The intraarticular environment of the knee is not conducive to ligament healing due to the presence of synovial fluid. Attempts at supportive care to promote anterior cruciate ligament (ACL) healing have a substantially high rate of failure (40% -100%), even after surgical repair using suture. Synovial fluid provides necessary nutrition and lubrication to articular cartilage and also presents an obstacle to ligament healing. Polymer fibers made of poly(lactic acid) (PLLA), polyglycolic acid, and polylactic-co-glycolic acid can be wound into scaffolds and implanted surgically using the same techniques as an allograft or autograft. These polymer scaffolds, especially when combined with biologics such as bone morphogenetic protein and other growth factors, provide soft tissue reconstruction solutions for both articular cartilage and ligaments in the presence of synovial fluid.
The potential market for application of synthetic ACL grafts is growing. Current trends in the United States suggest that regenerative engineering advances in ACL reconstruction would be timely as between 1994 and 2006, the population-adjusted estimate of the rate of ACL reconstructions increased by 37% (33.0/100,000 capita or 86,837 total procedures to 45.1/100,000 capita or 134,421 total procedures). Synthetic ACL grafts would provide a real option for patients who prefer to not undergo tissue harvest and avoid the associated donor site morbidity associated with hamstring or patellar tendon harvest; who wish to avoid the small, but real, risk of disease transmission and higher graft failure rates associated with donor grafts (allograft); and those patients who require revision surgery due to previous ACL graft reconstruction failures in the setting of autografts or allografts.
While primary repair of torn anterior cruciate ligaments fell out of favor, the advent of scaffold has brought about a renewed interest in the ability to suture repair cruciate ligaments in the intraarticular milieu. Bridge-enhanced ACL repair (BEAR), involves suture repair of the ligament in the presence of a bioactive scaffold to bridge the gap between the torn ligament ends and facilitate healing. In a cohort study with 20 patients, 10 patients underwent BEAR and were compared with 10 patients who underwent reconstruction with hamstring autograft. There were no differences between groups in effusion or pain and no failures by Lachman examination criteria. Magnetic resonance images from all the BEAR and ACL-reconstructed patients demonstrated a continuous ACL or intact graft. Patients who underwent BEAR had significantly greater hamstrings strength at 3 months postoperatively than the autograft control group. The authors concluded that these promising results should lead to future studies with larger groups so more conclusive results can be obtained.
The Evolution of Materials and Their Application in Regenerative Engineering
The variety of materials that can be used to develop biologically compatible scaffolds capable of supporting bone growth provides a stage for what engineers can use to address tissue engineering challenges. Implant development no longer centers on how to create implants that are biologically inert but rather on how the implant can impart the desired biological effect on the body.
When implants and scaffolds are placed in an organism, they must survive a highly corrosive environment for sufficient time for tissue ingrowth and maturation. As a simplification, scaffolds generally are broken into categories of synthetic polymers, natural polymers, metals, ceramics, and composites. Each material has its benefits and challenges. Metals in the corrosive environment can react and corrode, creating inflammation and ultimately destroying surrounding tissues. Polymers can fatigue and fail as they do not undergo remodeling as natural tissues in the body. Ceramics can be brittle and fracture. The use of composite materials and the introduction of three-dimensional printing provide new methods to overcome the shortcomings of earlier scaffold materials and designs.
A good example of the evolution of a material is the use of ceramics. Used first in implants due to their biologic inertness, first-generation ceramics included alumina, zirconia, as well as porous ceramics whose physical structure facilitates bone ingrowth. Alumina, in the form of alpha-Al 2 O 3 , was first reported as a component of a femoral head in a joint arthroplasty in 1972. Ceramic materials were later used for acetabular cups. Ceramics make good implant materials as they have low wear rates, resistance to corrosion, high strength, and good biocompatibility when compared with previous acetabular cups made of polyethylene. Despite all these favorable characteristics and improved biocompatibility when compared to polyethylene which shed particles, which would result in osteolysis, ceramic acetabular cups had low fracture toughness resulting in catastrophic failures. Currently, ceramics such as alumina and zirconia are used for total joint arthroplasty in conjunction with other types of materials such as ceramic femoral heads with polyethylene liners.
None of those early designs really took advantage of ceramics as a scaffold until the modification of surface characteristics on the implant to improve bone ingrowth. In addition, porous ceramics intuitively would provide an opportunity for native tissues to integrate into the structure of the scaffold. Alumina and zirconia can be made into porous forms with foaming agents that produce gases while the ceramic is setting, resulting in relatively diffuse and random distribution of pores. These porous ceramics could then be implanted in the hopes that surrounding osteocytes and fibroblasts would occupy those gas-created spaces and whiten the ceramic, integrating the ceramic into the surrounding bone. These experiments, however, led to disappointing results as the risk of mechanical collapse. One of the problems with porous ceramics is that they were too brittle and would fail catastrophically in a predictable fashion under stress. This does not happen in normal bone because the combination of inorganic HA (approximately 70%) and organic components such as collagen (approximately 30%) adds elasticity and flexibility.
Scientists also looked at nature for inspiration, where hierarchal patterns are common. Bone has two characteristic patterns, dense cortical bone as well as trabecular (also referred to as cancellous or spongy bone). The relatively heavy cortical bone provides strength and is load bearing. Human mandibular cortical bone has a porosity of approximately 3.5%. Its thickness and density varies with the amount of force that is imparted on it, referred to as Wolff’s law with higher load–bearing bones such as the femur having thicker and denser cortical bone than the upper extremities. In addition to being responsive to mechanical forces, the cortical bone also has a complex microstructure including canals and conduits for blood supply, innervation, and cell-to-cell communication between osteocytes, as well as providing specialized pores for cells involved in bone turnover such as osteoblasts and osteoclasts.
Trabecular bone with its honeycomb-like structure is contained within the cortical bone shell. Trabecula (plural trabeculae, from Latin for “small beam”) is a small, often microscopic, tissue element in the form of a small beam, strut, or rod. The rod and beam structure of trabecular bone, in contrast to the denser cortical bone, creates a lightweight network of cavities within the bone. Human mandibular trabecular bone is less dense with porosity of about 79.3%. This reflects its function in the body. The trabecular bone does contribute to the overall strength of the bone, and it also provides space for both fatty and red bone marrow as well as blood cell production.
Looking elsewhere in nature for hierarchal structures that could dissipate forces, scientists noticed that the calcium carbonate structure of certain corals mimic trabecular bone. Beginning in the 1970s scientists began exploring the use of natural coral graft substitutes derived from the exoskeleton of marine madreporic corals as ostoconductive bone xenograph. The porite, which is the structural unit of coral, is similar to that of cancellous bone, both in their scaffold-like network of channels and cavities as well as some of their mechanical properties. Made up of primarily calcium carbonate, treated coral proved to be biocompatible being relatively inert like many other ceramics, osteoconductive providing channels for blood vessel ingrowth similar to trabecular bone, and bioresorbable over time. To minimize and prevent bioresorbability for creating a longer lasting implant, coral can be treated using a hydrothermal process to convert it to a nonresorbable HA material. However, the hierarchal structure of corals, while mimicking native trabecular bone, did not have osteoinductive or osteogenic properties unless combined with other biologics. Coraline matrix is currently sold as calcified matrix for use in orthopedic surgery.
Another source of calcium minerals from nature for bone scaffold is avian eggshell. Similar to both bone and coral, eggshell is composed primarily of calcium and phosphate. Although eggshell as a natural biomaterial is biodegradable, readily available, and inexpensive, it requires surface modification to improve cell interactions. These modifications include hydrothermal treatment of eggshell to calcium-deficient HA with surface microstructure. This resulted in higher osteoblast viability on surface-modified ES than a commercially available bone substitute, bovine bone (Bio-Oss, BO). In in vivo studies, there was significantly greater new bone formation and mineralized bone-to-graft contact of surface-modified ES, especially with hydrothermally treated ES, compared with BO in 5-mm diameter calvarial defects in rats at 4 and 8 weeks of healing on both histological and histomorphometric analysis. In 2011, scientists synthesize 3D calcium carbonate interwoven nanofibrous platforms from eggshells using high-repetition femtosecond laser irradiation. This was the first study looking at synthesizing 3D nanofibrous structures from eggshell. Three-dimensional (3D) nanostructures are preferred to act as growth-support platforms for bone and stem cells.
While coral and eggshell, both calcium-based natural bioceramics, seem intuitively modifiable to form bone scaffolds, another source of natural xenograft is, surprisingly, trees. In 2009 Italian scientists announced a breakthrough in the use of wood as a bone substitute. Trees and other vascularized plants contain as hierarchal microstructure consisting of channels which bring water and sap up and down the organism through canals called xylem and phloem. Similar to bone, these channels are organized in a hierarchal fashion. A HA scaffold can be made from native wood through a sequence of thermal and hydrothermal chemical processes. These reactions, though highly caustic, ultimately yield calcium carbonate (the same component in untreated coraline xenografts) and then undergo phosphatization through hydrothermal treatment to achieve HA. This yields an inorganic biomorphic scaffold providing a biomimetic nanostructure surface for orthopedic and engineering applications. The inventors of this wood-derived, HA scaffold claim that their material will permit better penetration during bone growth and allow more flexion than metal or hard ceramic grafts. This demonstrates that scientists can look to nature for inspiration and ways to repurpose materials that evolved to serve other purposes.
Scientists no longer have to be limited to random pattern ceramics through chemical reactions or rely on nature to repurpose other hierarchal patterns into scaffolds. Three-dimensional printing (3DP) can overcome the limitations of using natural tissues and open up the possibility of high-precision microstructural engineering of materials to take advantage of the physical characteristics of novel hierarchal designs and patterns. Take for example, our discussion of avian eggshell nature-derived scaffolds. The eggshells could be crushed into a powder and then pressed into blocks to be used in their native form as bone scaffolds, or 3DP could be used to construct the biomaterial into a new structure altogether. Eggshell was explored as a precursor for the biologically active bone implant. Park et al. fabricated 3D-lattice-patterned scaffolds from slurry created from crushed Gallus gallus eggshells via coagulation-assisted extrusion-based printing. Notably, compared to contemporary methods, a multiphasic CaP-based 3D scaffold with hierarchical porosity was prepared using a simple slurry processing, followed by a single heat-treatment cycle. When compared to a similar scaffold fabricated from a chemically synthesized calcium phosphate powder, the naturally sourced eggshell scaffold had improved biological activity in comparison with the synthetic one. Enhanced cell activity promoted significant ECM deposition during in vitro biological assessment as well as higher cell adhesion and rapid differentiation into osteogenic lineage. In sense, the use of 3DP enhanced and modified the biologic activity that the original material imparts on the surrounding host cells.
This theme of remanufacturing and redesigning existing materials has been the focus of Markus Buehler’s group of the Department of Civil and Environmental Engineering at the Massachusetts Institute of Technology. “Metamaterials” is the term coined by this group for creating new composites with novel, emergent properties not found in their component materials. This group integrates advanced design, computational modeling, and 3-D assembly with chemistry, materials engineering, and tissue engineering to generate entirely new “metamaterials” that are then printed into novel structures using a 3D printer. In other words, materials can be arranged into new structures to generate scaffolds that today do not exist in either engineered or biological forms. These metamaterials can be chemically or physically resurfaced to interact and direct the growth of surrounding tissues, degrade at predetermined rates, have complex structures including channels and pores to direct the development of structures such as blood vessels and nerves, and have specific physical and mechanical behaviors.
In 2013 Mueller’s group published a novel, 3DP composite material that consisted of two materials, one with high compliance, and the other highly brittle, printed into three distinct biologically inspired patterns. These patterns included a bone-like pattern, a biocalcite pattern, and a rotated bone pattern. From the base materials that are brittle and exhibit catastrophic failure, synthetic composites are created with superior fracture mechanical properties exhibiting deformation and fracture mechanisms reminiscent of mineralized biological composites. The fact that the experimental fracture behaviors resembled the computer models of failure also indicated that computer models to design composite materials to exhibit tailored fracture properties and then use 3D printing to synthesize materials with such mechanical performance.
The scope of novel designs and composite materials becomes possible by combining computer design, microengineering, and 3DP may be essential for the fabrication of scaffolds capable of inducing complex tissue regeneration such as in organ or limb regeneration. This would include the growth of gradient tissues and structures such as joints which are made up of multiple types of tissue. Cell- and growth factor–based tissue engineering, especially if those signals can be geographically localized by binding signaling molecules to materials in predetermined specific locations yielding “intelligent” scaffolds that can direct the growth and behavior of the surrounding native tissue. This can include stimulating ingrowth of the surrounding native tissue and directing the development of local stem cells in the local native tissue to yield new tissue types distinct from the surrounding tissues.
Some of the ways scaffolds can be fabricated to direct the propagation, migration, and differentiation of surrounding cells by modifying their surface characteristics, their ultrastructure in the case of nanofibers, or by the inclusion of bound growth factors and biologics. Growth factors that have been surface adsorbed or encapsulated in scaffolds include vascular endothelial growth factor (VEGF), platelet derived growth factor (PDGF), epidermal growth factor (EGF), and bone morphogenic protetin (BMP). These scaffolds can then trigger specific biological processes such as angiogenesis, bone formation, and fibroblast ingrowth. The method of cross-linking or embedding growth factors onto or into scaffolds can control the timing of their release and thus the biological signal into surrounding tissues.
Similar to biologics, physical factors such as the ultrastructure of a scaffold can influence cell behavior, just as dramatically as signal transduction and growth factors. Advances in textiles and materials science have given rise to electrospinning as a fiber and scaffold production method which uses electric force to draw charged threads of polymer solutions or polymer melts up to fiber diameters in the order of some 100 nanometers. Working with polymers of this scale creates a very large surface area to volume ratio (this ratio for a nanofiber can be as large as 103 times of that of a microfiber), increase flexibility in surface functionalities, and superior mechanical performance (e.g., stiffness and tensile strength) compared with other materials and scaffolds even made with the same type of polymer.
These fibers can then be further woven into scaffolds in the form of patches, meshes, tubes, or cords with precise and complicated microarchitecture structures that in themselves can influence cell behavior. These nanofiber scaffolds can be so finely engineered so that their morphological is similar to the native ECM. Nanoscale alterations in scaffold topography elicit diverse cell behavior, ranging from changes in cell adhesion, motility and migration, activation of specific signal transduction cascades such as tyrosine kinases, and even lead to upregulation or downregulation of gene expression. For example, skeletal muscle can be cultured in vitro; however, if the fibers are not oriented in a systematic fashion, the resulting tissue will not be functional. A nanoscale polycaprolactone scaffold can be constructed and seeded with myoblasts which then orient themselves in a uniform pattern as well as change their morphology with a larger length to diameter ratio which is compatible with force production characteristic of skeletal muscle tissue.
Nanofibers can also be conductive of physical forces and charges. Piezoelectric effect is the ability of certain materials to generate an electric charge in response to applied mechanical stress. The word Piezoelectric is derived from the Greek piezein, which means to squeeze or press, and piezo, which is Greek for “push.” Bone tissues have the ability to generate electrical signals. This bioelectrical current is generated by the shear force of collagen and the deformation of fluid-filled channels within the bone. The stress-generated electrical stimuli might play a determining role in impacting osteogenic growth.
In orthopedics, the use of electric and electromagnetic fields has focused primarily on promoting healing of bony nonunions. There are multiple strategies for applying electrical forces across nonunions and bony deficiencies. Direct current (DC) involves the surgical implantation of a cathode at the fracture site and an anode in the nearby subcutaneous tissue, with the production of an electric current between them. Pulsed electromagnetic fields (PEMFs) therapy is a noninvasive mode of bone-growth stimulation involving placement of a wire coil over the fracture site. Capacitive coupling (CC) is where the electrical field is created by an oscillating electric current produced between two capacitor plates placed on the skin on opposite sides of the fracture. Finally, combined magnetic fields (CMFs) are produced through the combination of alternating and DC electric stimulation and are delivered by an external pair of coils applied over the fracture site which is worn by patients for 30 min a day.
Similarly, certain nanofiber scaffolds, by their ability to conduct charges, can affect the bone marrow stroma, which consists of a heterogeneous population of cells that provide the structural and physiological support for hematopoietic cells. The bone marrow stroma also contains precursor cells, with stem cell–like character, which can differentiate into bone, cartilage, adipocytes, and hematopoietic supporting tissues. In addition to hypoxia and growth factors as development signals to differentiate cells within the bone marrow stroma, electrical stimulation (ES) is known to be able to promote osteogenic differentiation of bone marrow storm cells (BMSCs).
External stimulation, in the form of magnetic fields, electrical currents, or electromagnetic forces, is known to play a role in articular cartilage maintenance and regeneration as well as in bone remodeling and enhances bone healing. This effect can also be enhanced by nanofiber scaffolds, and this effect opens the door for bone healing strategies that take advantage of both external stimulation and smart scaffold design. In one study, to enhance the local electrical stimulation effect on surrounding BMSCs, parallel-aligned conductive nanofibers were electrospun from the mixtures of poly( l -lactide) (PLLA) and multi-walled carbon nanotubes (MWCNTs) and used for cell culture. Cultured BMSCs were exposed to ES (direct current, 1.5 V; 1.5 h/day) applied perpendicular to the nanofiber scaffold fibers within the BMSC culture. They found that in comparison with ES-free groups, bone-related markers and genes were found significantly upregulated when ES was applied on BMSCs growing on nanofibers having higher conductivity, especially when the ES signal was applied earlier in the stem cell differentiation. Similar results were observed in a rat calvarium model in which polarized nanocomposite membranes were applied to 5-mm calvarial defects in 8-week-old male rats. Each rat underwent the creation of two calvarial defects, with the right-sided defect implanted with electroconductive scaffold and the left undergoing implantation with nonconductive scaffold as a control. Micro-CT analysis showed that the polarized nanocomposite membranes lead to an increase in the amount of regenerated bone volume and bone mineral density, and the nascent bone was indistinguishable from the surrounding host bone after 12 weeks implantation of polarized nanocomposite membranes when imaged with X-rays.
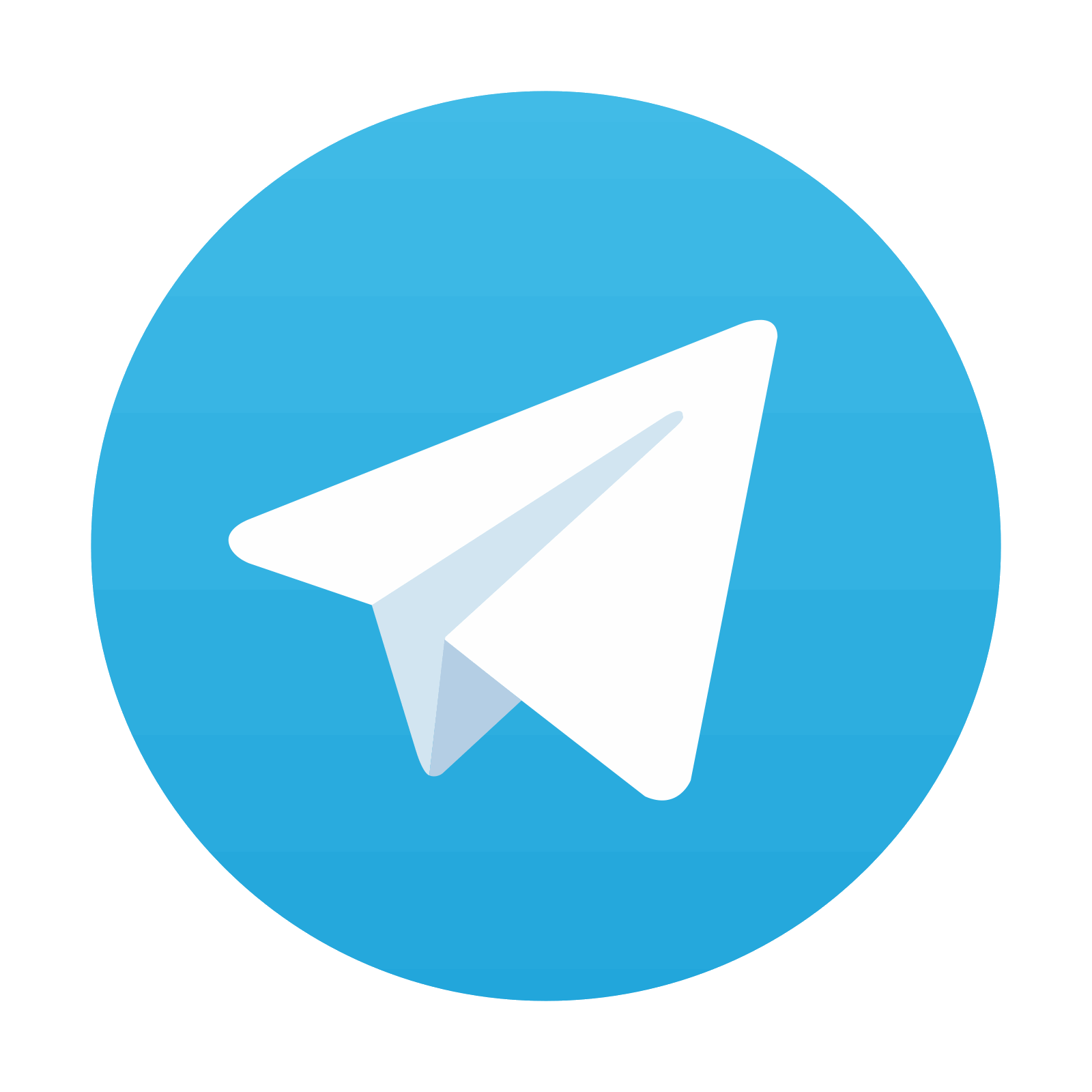
Stay updated, free articles. Join our Telegram channel
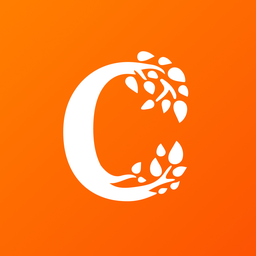
Full access? Get Clinical Tree
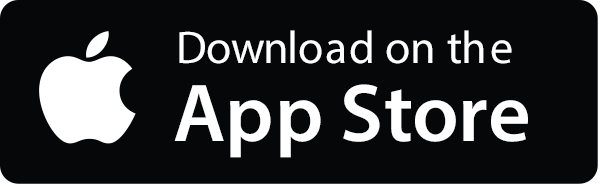
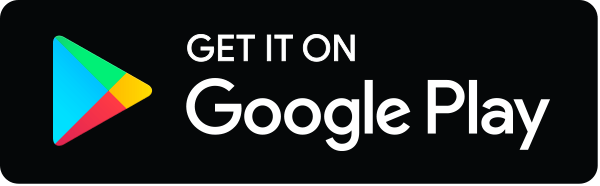