Abstract
A cell is the most fundamental unit of a living organism. For the most part, a cell is a small membrane-enclosed structure that consists of an aqueous solution of cytoplasm that is filled with an abundance of biomolecules, such as proteins and nucleic acids. Along with this composition, a cell is the smallest unit of a living organism that is able to independently replicate itself. As a result, humans are a product of multiple cell divisions, all from a single cell, that ultimately coalesces into more than 10 13 cells that form the human body (Alberts, 2015; Elabd et al., 2007). 1,2 The replication potential of human cells, taken together with the composition of cells, have gained widespread interest as agents to be harvested from humans and used to accelerate and amplify the biology of healing. Cell therapy involves the harvest of cells, with therapeutic potential, and then the delivery of these isolated cells to the pathologic area of injured muscle, tendon, ligament, or bone. For this reason, this chapter will discuss the use of cells to augment biologic healing in musculoskeletal conditions. Specifically, we will review the use of bone marrow aspirate concentrate, autologous chondrocyte implantation, and stem cells as a source of cellular-based therapies in commonly encountered conditions in orthopaedics.
Keywords
Autologous chondrocyte implantation, Bone marrow aspirate concentrate, Mesenchymal stem cells, Stem cells
Bone Marrow Aspirate Concentrate
Bone marrow aspirate concentrate (BMAC) is derived from fluid obtained from bone marrow. Given the source of origin, BMAC contains a composition of cells—mesenchymal stem cells (MSCs), hematopoietic stem cells, endothelial progenitor cells, white blood cells, and red blood cells. Equally important, BMAC, along with a strong source of cells, also contains platelets, cytokines, and growth factors such as bone morphogenic proteins (BMPs), platelet-derived growth factor (PDGF), transforming growth factor-B, vascular endothelial growth factor (VEGF), interleukin-B, and interleukin-1 receptor antagonist ( Fig. 4.1 ). This combination of cells and growth factors from BMAC allows a single source to support cell growth and recovery following injury to musculoskeletal tissue.

The Importance of Concentrating Bone Marrow Aspirate
Without concentration techniques, bone marrow aspirate alone has a low percentage of MSCs of only 0.001%–0.01% of nucleated cells. For this reason, centrifugation is used to concentrate bone marrow aspirate in order to increase the percentage of MSCs. In bone marrow aspirate, the predominant cell type is neutrophils and erythrocytes. With this in mind, there have been gender differences noted in the predominant cell type among bone marrow aspirate with male aspirate containing more erythroblasts and female aspirate containing more neutrophils. In general, the cellular composition of BMAC is 28.1% erythroblasts, 32.7% neutrophils, 13% lymphocytes, 2.2% eosinophils, 1.3% monocytes, and 0.1% basophils. In comparison to platelet-rich plasma (PRP), the cellular composition of BMAC has 11.8 times the amount of white blood cells, 19.4 times the amount of neutrophils, and 2.5 times the amount of platelets. Similar to PRP, BMAC contains a comparable concentration of monocytes, lymphocytes, eosinophils, and basophils.
The importance of concentrating bone marrow aspirate into BMAC has been highlighted by several studies supporting a direct correlation of the number of cells with healing potential and optimal outcomes. With this in mind, the minimum number needed, to induce biologic healing, is 1000 progenitors/cm 3 of BMAC. In like manner, a study on bone healing of nonunions showed a statistically significant lower rate of union in patients who received a BMAC with less than 1000 progenitors/cm 3 and less than 30,000 progenitor cells in total. At the same time, this study found a positive correlation between the number and concentration of osteoprogenitor cells delivered in the bone marrow aspirate with the volume of mineralized callus present at the sites of bone healing. All things considered, Hernigou et al. concluded that 1 mL of bone marrow aspirate contained a competent concentration of cells to form 1 cm of bone in areas of nonunion. Further emphasis has been placed on the critical concentration of certain cellular elements, such as MSCs, rather than the total cell count of the entire composition of cells contained within BMAC. As can be seen, the concentration of bone marrow aspirate is imperative to obtain the optimal volume of the essential cellular elements, i.e., MSCs, recommended to expedite the biologic healing response; yet, BMAC also provides a source of growth factor therapy to enhance treatment.
Growth Factors and Cytokines Contained in Bone Marrow Aspirate Concentrate
Growth factors are biologically active polypeptides that can be applied to stimulate cell growth and enhance both chondrogenesis and osteogenesis. Commonly encountered growth factors, pertinent to healing of musculoskeletal tissue, are transforming growth factor-β2 (TGF-β2), PDGF, fibroblast growth factors (FGFs), VEGF, interleukins (ILs), Insulin-like growth factor-1 (IGF-1), and BMPs ( Table 4.1 ). BMAC, not to mention, has an increased concentration of growth factors due to the substantial number of platelets with α-granules. Specifically, the α-granules of platelets contain TGF-β2, PDGF, VEGF, FGF, BMP, and IGF. In comparison to PRP, BMAC has a significantly increased supply of growth factors with 172.5 times more VEGF, 78 times more IL-8, 4.6 times more IL-1B, 3.4 times more TGF-β2, and 1.3 times more PDGF. Each of these aforementioned growth factors has specific functions to stimulate healing in select tissues important in orthopaedics. As an illustration, the most common growth factor used to stimulate chondrogenesis is TGF-β2, which stimulates extracellular matrix synthesis and chondrogenesis in the synovial lining all while decreasing the catabolic activity of IL-1. Explicitly, the proliferation and differentiation of chondrocytes by TGF-β2 is via phosphorylation of SMAD (Suppressor of Mothers Against Decapentaplegic) proteins that transduce extracellular signals to the nucleus to active downstream gene transcription for chondrogenesis. In the same fashion, BMPs have been shown to stimulate, through autocrine signaling, both osteogenesis and chondrogenesis. In particular, BMP-2 has been used to stimulate bone growth in the setting of fracture healing, while BMP-7 has been used to stimulate cartilage matrix synthesis by inhibiting catabolic factors of matrix metalloproteinases. ILs, involved in the initial inflammatory response to injury, aid in cell migration to the site of injury while increasing production of other favorable factors, such as VEGF. In the same fashion, VEGF promotes angiogenesis to provide transport of necessary products for healing and growth of mesenchymal tissue. Further healing is augmented with PDGF as a chemotactic factor for mesenchymal cells and suppressor of IL-1β, which induces cartilage degradation by downregulation of nuclear factor-κβ. PDGF, at the same time, stimulates wound healing and collagen synthesis and promotes formation of cartilage. Another growth factor, commonly contained in BMAC, is IGF-1 that increases collagen and proteoglycan synthesis, increases metabolic activity, and helps maintain the integrity of articular cartilage. A large family of growth factors is FGFs with special emphasis for FGF-2 and FGF-18, both of which are involved in chemotaxis of MSCs and chondrogenic differentiation. Taken together, the growth factors and stem cells contained in BMAC are individually important in augmenting the biologic healing of musculoskeletal tissue.
Growth Factor/Cytokine | Function |
---|---|
TGF-β1, TGF-β2, TFG-β3 | Chondrogenesis via SMAD |
PDGF | Wound healing, collagen synthesis, enhanced BMP signaling, promotes cartilage formation |
FGF-2, FGF-18 | Chondrogenic differentiation, MSC chemotaxis |
VEGF | Angiogenesis, supports bone/cartilage growth |
IL-1, IL-8 | Inflammatory response, MSC chemotaxis to injury site |
IGF-1 | ↑ metabolism, ↑ proteoglycan synthesis, ↑ collagen synthesis |
BMP-2 | Osteogenesis/chondrogenesis, matrix synthesis |
BMP-7 | Cartilage matrix synthesis, ↑ extracellular matrix |
Sources of Bone Marrow Aspirate
The aspiration of bone marrow can be performed at various locations throughout the human body. Despite the different anatomic locations available for bone marrow aspiration, all sites of aspiration do not provide the same proportion of BMAC. In fact, the quantity of progenitor cells, from bone marrow aspirate, decreases from axial to appendicular skeleton as well as from proximal to distal in the appendicular skeleton. The highest quantity of progenitor cells in bone marrow aspirate has been found in the vertebral bodies. Although this may be true, aspiration of bone marrow from the vertebral body is not as practical as other available anatomic sites for aspiration. More reasonable locations for aspiration have been obtained from bone near the site of surgery or the iliac crest, which is based on both accessibility and percentage yield of progenitor cells. In like manner, a study comparing sites to harvest BMAC from the iliac crest, tibia, and calcaneus found that the highest concentration of progenitor cells resided in the iliac crest. Equally important, this study demonstrated that all anatomic sites of harvest, even the proximal tibia and calcaneus, are both safe and amenable to achieve adequate amounts of progenitor cells. By the same token, no difference in cell yield was noted with demographics of age or sex or with comorbidities of smoking and diabetes. Given the accessibility of the iliac crest, this anatomic site is one of the most commonly used locations for obtaining bone marrow aspirate. For this reason, Pierini and colleagues further evaluated the yield of progenitor cells from either the anterior or posterior iliac crest. In comparison to both sites of the iliac crest, the highest yield of colony-founding connective tissue progenitor cells was aspirated from the posterior iliac crest. However, no difference in the biologic potential in terms of viability or differentiation of MSCs was noted between the anterior or posterior iliac crest. In spite of this study, another study by Marx and colleagues found no significant difference in the yield of either stromal stem cells or hematopoietic stem cells between the anterior or posterior iliac crest. Rather, both the anterior and posterior iliac crest harvest provided twice the amount of stromal and hematopoietic stem cells than harvest from the tibial plateau. As can be seen, the iliac crest is the preferred anatomic location for aspiration of bone marrow, yet other anatomic sites, near the area of surgery, may be able to provide the principal BMAC preparation required for healing.
The Bone Marrow Aspirate Concentrate Technique
The technique of harvesting BMAC can be applied to most anatomic locations. For simplicity, the technique will be further described in reference to the iliac crest, which is the most commonly used site for aspiration of bone marrow. The technique begins with a percutaneous stab incision placed over the iliac crest. Without delay, a Jamshidi needle is directed parallel to the iliac wing between the inner and outer tables of the crest. With use of the Jamshidi needle two starting holes are made, with one hole used to collect the first 30 mL of aspirate and the second hole used to collect the second 30 mL of aspirate. To begin with, 5 mL of marrow is aspirated, and then the Jamshidi needle is rotated 45 degrees to begin the next aspiration. After the next 5 mL of bone marrow is aspirated, the Jamshidi needle is advanced 1–2 cm, and then the process is repeated with another 5 mL aspiration followed by a 45-degree rotation of the needle. With each advancement and reposition of the needle, the obturator is reinserted into the needle in order to clear the bore of the needle from debris. Importantly, the vacuum aspiration of bone marrow occurs in conjunction with anticoagulation via heparin/ACD-A solution (8 mL of anticoagulant per 60 mL of bone marrow aspirate). In due time, with aspiration of the desired volume of bone marrow, the fluid is concentrated with use of a centrifuge system. Straightaway processing and concentration of bone marrow aspirate takes place with density gradient centrifugation using an automatic microprocessor-controlled centrifuge system. Following about 15 minutes of centrifugation, the erythrocytes, nucleated cells, and plasma are separated into a second chamber. Completion of the centrifugation process yields approximately 7–10 mL of BMAC available for injection into the designated area to augment biologic healing.
Several studies have investigated multiple methods to optimize the technique of harvesting bone marrow aspirate. These studies have evaluated the needle advancement, the syringe size, the aspirate volume, and the different commercial systems available for BMAC harvest ( Table 4.2 ). With attention to needle placement, movement of the needle throughout the aspiration process provides a higher yield than a stationary needle. Peters and colleagues demonstrated that multiple needle advancements resulted in a higher concentration of MSCs. In particular, needle advancement of 5 mm up to 3 times throughout the procedure increases the proportion of MSCs. Not to mention, the size of the syringe used for vacuum aspiration of the bone marrow matters in regard to cell yield. In a study by Hernigou et al., a 10 and 50 mL syringe were compared during bilateral aspiration of bone marrow from the iliac crest. In comparison, there was a 300% higher cell yield with a 10 ml syringe, and equally important, there were significantly more cells in the first 1 mL aspirate of the 10 mL syringe as opposed to the first 5 mL of the 50 mL syringe. As a result, the more efficient technique to optimize aspiration of bone marrow is by aspirating smaller volumes in small syringes. By the same token, larger volumes of aspirate appear to dilute the concentration of bone marrow. As small a difference as 2 mL of bone marrow aspirate can produce a drastic difference in the amount of MSCs. Indeed, Muschler and colleagues demonstrated a difference between 2 and 4 mL of bone marrow aspirate from the anterior iliac crest of patients with the number of MSCs decreasing by 50% from the higher volume of aspirate. In like manner, a study by Bacigalup et al. determined that harvest of bone marrow using multiple small volume aspirations decreased the dilution with peripheral blood, thus resulting in a higher yield of MSCs. Multiple commercial companies provide systems for harvesting of bone marrow aspirate. A study comparing different systems to harvest BMAC included the following systems: Harvest SmartPRep2, Biomet BioCUE, and Arteriocyte Magellan. In comparison of these systems, the Harvest system showed significantly greater concentration of connective tissue progenitors both before and after centrifugation in comparison to the Biomet BioCUE system. In a similar fashion, the Harvest system showed significantly higher percent yield of connective tissue progenitor cells after centrifugation in comparison to the Arteriocyte Magellan system. Under these circumstances, the difference between systems, in harvest of bone marrow aspirate, is believed to be due to differences in the centrifugation device that leads to a variation in yield of concentrated progenitor cells.
Technique Optimization | Comment |
---|---|
Location | |
|
|
Volume | |
|
|
Needle Placement | |
|
|
Centrifugation System | |
|
|
The Application of Bone Marrow Aspirate Concentrate
The utility of BMAC has been applied to address numerous pathologies of the musculoskeletal system. As an illustration, BMAC has been used to induce and increase bone formation. In an analysis of basic science evidence for long bone healing, Gianakos et al., summarized that several studies support BMAC significantly increasing the amount of bone formation. To demonstrate this finding, imaging showed significant increases in bone formation by an increase in bone volume, callus formation, and union of healing bone. At the same time, on microcomputed tomography of animal subjects receiving BMAC, there was an 81% reported significant increase in the bone area of these study subjects. Under closer observation, histologic and histomorphometric assessment of BMAC subjects showed a 90% significant improvement in earlier bone healing. The increased bone formation, in bone defects treated with BMAC, translated into a higher torsional stiffness of 78%. In like manner, BMAC, in addition to adding bone, has also exhibited the ability to add cartilage and augment cartilage healing. Must be remembered, BMAC contains MSCs that have been validated to increase aggrecan content and tissue firmness that together compliment the cartilage repair. In a goat model by Saw and colleagues, surgically created cartilage defects when treated with BMAC, along with hyaluronic acid (HA), promoted the healing of cartilage with more glycosaminoglycan and a higher content and a better organization of hyaline cartilage. In an equine model by Fortier and colleagues, cartilage defects were treated with either microfracture alone or microfracture along with BMAC. In comparison of both groups, the addition of BMAC resulted in increased glycosaminoglycan, increased type II collagen, improved collagen orientation, and improved filling of cartilage defects. Important to realize, the remarkable results in animal models has translated into optimized outcomes in human patients. In a study by Gobbi et al., BMAC was compared with matrix-induced autologous chondrocyte implantation (MACI) for treatment of patellofemoral chondral lesions. Although both groups of patients reported significant improvements, patients treated with BMAC showed significantly greater improvements in functional outcome scores. Summation of clinical studies of BMAC, through a systematic review by Chahla et al., has synthesized good to excellent overall outcomes with treatment of BMAC for both knee osteoarthritis and knee chondral defects. Another key point, from this systematic review, was that BMAC has better outcomes for treatment of cartilage lesions in patients younger than 45 years, with smaller size of chondral lesions, and with a fewer number of lesions. Even though there is a broad range of clinical applications for BMAC from healing of fractures to treatment of osteoarthritis or cartilage defects, future studies are warranted to further elucidate the exact mechanisms and particular patients that would most benefit from augmentation of biologic healing with BMAC.
Safety of Bone Marrow Aspirate Concentrate
As with all treatment inventions, the safety and risk of potential complications must be weighed against the benefits of the BMAC procedure. The use of BMAC requires harvesting the bone marrow from a donor site of the patient; this entails an additional area of interruption and potential increased morbidity from the surgical technique. For fear of morbidity, the safety of autologous BMAC was evaluated by Hendrich et al., in the initial experiences in 101 patients. After an average of 14 months, upon reexamination of all the patients, no major morbidity or mortality was directly due to the BMAC treatment. In particular, there were no noted infections, no induction of tumor formation, and no noted complications at the harvest site of the iliac crest. Given the contents of BMAC containing stem cells, there is concern of cancerous formation from these pluripotent cells. With this in mind, the risk of cancer was explored by Hernigou et al., in patients treated for orthopaedic diseases with autologous bone marrow cell concentrate. At a mean of 12.5 years of follow-up, there was 1873 patients treated with BMAC, and after reviews of more than 7300 magnetic resonance images (MRIs) and 52,000 radiographs, there were only 53 cancers diagnosed and not one of these cancers occurred in areas of the body that received an injection of BMAC. On a less severe scale of complications, one of the most common adverse events reported, after BMAC treatment, were swelling and pain at either the harvest site or the site of injection. As an illustration, these common complications of swelling and pain were noted in the majority of the 75 degenerative knees that received BMAC for osteoarthritis. On the contrary, in another study by Centeno and colleagues, after 840 BMAC procedures for knee osteoarthritis, no severe adverse events post procedure were due directly from the BMAC technique. In detail, symptoms after BMAC treatment were 6% for those patients who only received BMAC and 8.9% for those patients who received BMAC with adipose graft with the majority of these symptoms being mostly self-limited pain and swelling. As a result, the consensus of these studies conclude that BMAC treatment has no significant increase risk of cancer, limited harvest site morbidity, and the most common complications being self-limited symptoms without severe consequence.
Chondrocyte Implantation
Articular cartilage, one of the five forms of cartilage, functions to decrease friction and distribute loads across a joint. The composition of cartilage is cells, primarily chondrocytes, and extracellular matrix, with the majority of the matrix consisting of water and type II collagen. Of course, this composition of articular cartilage is arranged into three zones and a tidemark, which are established by the shape of the chondrocytes and the orientation of the type II collagen ( Fig. 4.2 ). In correspondence, the three zones are the superficial tangential zone, the intermediate zone, and the deep basal zone ( Fig. 4.2 ). In the first place, the superficial tangential zone contains flattened chondrocytes with the type II collagen orientation parallel to the joint to resist shear forces. The next zone, intermediate zone, is the thickest layer with round chondrocytes and type II collagen oriented oblique to the joint. The last zone, deep basal zone, contains round chondrocytes with type II collagen orientation perpendicular to the joint to resist compressive forces. Below the deep basal zone is the tidemark that divides the superficial uncalcified cartilage from the deeper calcified cartilage. Perforation of the tidemark, such as with an awl in microfracture, allows marrow elements to extricate and eventually end up filling the cartilage defects as fibrocartilage.

Injury to Articular Cartilage
Articular cartilage is an avascular structure that receives nourishment at the surface from synovial fluid. Given the avascular nature of articular cartilage, there is limited ability for healing of defects created in the surface. Furthermore, the number of chondrocytes in the articular cartilage is limited, and these chondrocytes have limited migratory ability that together further limit the healing potential of cartilage. Often, injury to the articular cartilage occurs from acute high-impact loading to a joint or from repetitive shear and torsional loads to the superficial zone of the articular cartilage. When this injury penetrates past the tidemark into the underlying subchondral bone, MSCs, through a bleeding response, fill the injured area of articular cartilage with fibrocartilage. However, fibrocartilage possesses different properties than articular cartilage with a higher coefficient of friction. To help understand the severity of injury to the articular cartilage and to help guide treatment for defects to this surface, classification systems, with the most commonly referenced being the Outerbridge grading system and the International Cartilage Repair Society (ICRS) grading system, have been established ( Table 4.3 ). In accordance, the Outerbridge arthroscopic grading system consists of the following grades: grade 0—normal cartilage; grade I—softening and swelling; grade II—superficial fissures; grade III—deep fissures without exposed bone; grade IV—exposed subchondral bone. In a similar fashion, the ICRS grading system follows the following format: grade 0—normal cartilage; grade 1—superficial lesions; grade 2—lesions <50% of cartilage depth; grade 3—lesions >50% of cartilage depth; grade 4—lesions extending into subchondral bone. With these classifications systems in mind, the proper indications for patient treatment selection and the prevalence of cartilage injuries can be communicated.
Outerbridge Grading System | International Cartilage Repair Society (ICRS) Grading System | |
---|---|---|
Grade 0 | Normal cartilage | Normal cartilage |
Grade 1 | Softening/swelling | Superficial lesions |
Grade 2 | Superficial fissures | Lesions <50% cartilage depth |
Grade 3 | Deep fissures without exposed bone | Lesions >50% cartilage depth |
Grade 4 | Exposed subchondral bone | Through subchondral bone |
Injury to the articular cartilage, especially in the knee, is common. In a retrospective review of 31,516 knee arthroscopies, the prevalence of chondral lesions was 63%. In particular, grade III lesions of articular cartilage were most common on the patella and grade IV lesions were most common on the medial femoral condyle with an average of 2.7 lesions per knee. In another study of 1000 consecutive knee arthroscopies, the prevalence of chondral or osteochondral lesions were found in 61% of patients. By location, the main injury to the articular cartilage was found on the medial femoral condyle in 58%, patella in 11%, lateral tibia in 11%, lateral femoral condyle in 9%, trochlea in 6%, and medial tibia in 5% with an overall average area of injury to the surface of 2.1 cm 2 . The size of the chondral defect helps guide treatment approaches to address the articular cartilage. For contained cartilage lesions less than 2 cm 2 , an acceptable initial procedure is microfracture, such as that described by Steadman, where an awl is used to penetrate pass the tidemark into subchondral bone in order to release MSCs that eventually form fibrocartilage. For contained cartilage lesions greater than 2 cm 2 , but less than 12 cm 2 , an acceptable procedure to perform is autologous chondrocyte implantation (ACI). Indeed, ACI is ideal for treatment of ICRS grade 3 or grade 4 lesions, especially along the regions of the femoral condyle or the femoral trochlea. ACI is a staged procedure where the initial procedure involves a biopsy of articular cartilage that is followed by expansion of cultured chondrocytes that are then reimplanted in a second procedure to fill the defect in the surface of the cartilage. The technique of ACI was explored early in a rabbit model by Grande and colleagues, where autologous chondrocytes grown in vitro were labeled prior to grafting with a nuclear tracer then replanted into defects in the articular cartilage. From this implantation, 82% of the articular cartilage was found to be reconstituted and the nuclear labeled chondrocytes were found to be incorporated into the repaired matrix of cartilage. By all means, the evidence from the animal models led to translation of ACI used in humans. The initial experience of ACI in humans was reported by Brittberg et al., in 1994, where 23 patients with full-thickness cartilage defects had healthy chondrocytes from an uninjured area of the knee harvested, cultured, and then transplanted via injection into the injured area of cartilage. After transplantation, patients reevaluated with arthroscopy were found to have transplants with the same macroscopic appearance, as the surrounding healthy cartilage and biopsy of the transplanted area, under microscopic examination, showed hyaline cartilage.
The Autologous Chondrocyte Implantation Technique
The initial stage of the ACI technique requires obtaining a biopsy specimen from an uninvolved healthy area of articular cartilage. At the initial stage of the procedure, the defect in cartilage is closely evaluated for not only size of involvement but also for signs of any significant bone loss. If no subchondral bone is present in the area of the articular cartilage defect, then bone grafting may be required during this foremost part of the procedure. Also, sizing up the lesion allows the ordering of cells, prior to implantation, to be determined. Most commonly, the biopsy specimens are obtained from either the superomedial or superolateral edge of the femoral trochlea or from the lateral aspect of the intercondylar notch ( Fig. 4.3 ). In total, biopsy specimens should contain both the entire surface of articular cartilage as well as a small portion of the underlying subchondral bone. Total weight of the biopsy specimen should be 200–300 mg. Within this weight range, the biopsy specimen should contain between 200,000 and 300,000 cells. After obtaining the biopsy specimen, the harvested cells are maintained at 4°C until processing. Typically, one vial of cells is sufficient to supply distinct defects up to 6 cm 2 , assuming that, each vial contains approximately 12 million cells along with 0.3–0.4 mL of Ham F-12 medium with serum supplementation. Therefore, larger defects (>6 cm 2 ) or multiple defects may require additional vials; for this reason, determining the size of the cartilage lesion and presence of multiple defects in the cartilage should be closely observed and measured at the time of biopsy. During processing, the biopsy of cartilage is digested with enzymes with intention to release chondrocytes from the collagen matrix. For approximately 30 days, the chondrocytes are expanded in a suspension culture to obtain approximately 15–20 million cells.

In due time, the second stage of the ACI technique is performed to implant the cultured chondrocytes into the desired defect ( Fig. 4.4 ). Inaugural techniques of ACI were performed with a medially or laterally based mini arthrotomy. Subsequent and more recent generations of ACI techniques are performed, without arthrotomy, using only arthroscopic techniques. In certain instances, a one-stage application of minced articular cartilage may even be a potential procedure to perform. Regardless of the technical approach being performed, the defect in the articular cartilage has to be prepared prior to receipt of the cultivated chondrocytes. Preparation of the defect begins with debridement, back until normal vertical margins of articular cartilage are achieved. Any cartilage that is not normal, with delamination or defibrillation, is removed. During preparation of the graft bed, any bone deficiency greater than 6 mm requires grafting until an even and established graft bed is recreated. Care is taken during graft bed preparation to prevent penetration into the underlying subchondral bone in order to prevent hemorrhage throughout the implantation technique. If bleeding is encountered, potential options include using thrombin spray or 1:1000 epinephrine–normal saline solution mixture in cotton pledges to obtain hemostasis. To begin with, the first generation of ACI techniques used a periosteal graft to create a seal over the prepared defect, prior to injection of the chondrocytes. Normally, the periosteum consists of an outer fibrogenic layer and an inner cambium layer with the principal harvest site for the periosteal graft being the proximal–medial aspect of the tibia just distal to the pes anserinus or semitendinosus tendon insertion. Importantly, to preserve cells in the cambium layer of the periosteum, no electrocautery is used during the patch harvest. After obtaining the periosteum, the graft is secured in place, over the recipient bed, with 6-0 vicryl suture approximately 2 mm from the surface and extending peripherally approximately 4 mm from the defect edge. Once, each corner of the periosteal graft is tied down, additional sutures at about 3 mm increments are added around the graft to ensure a watertight seal. To detect any potential leak, prior to placement of the chondrocytes, normal saline can be injected under the graft to confirm an adequately sealed cover. At this instant, chondrocytes can be implanted under the watertight sealed periosteal graft, using about one vial per 6-cm-sized defect. After injection of the chondrocytes, fibrin glue is used over the periosteal graft to seal in the implanted cells.

The second generation, and more recent, techniques of ACI all involve a similar first step to obtain a biopsy specimen to be used for autologous chondrocyte expansion. Typically, the biopsy specimens, as has been noted, are collected from the non–weight-bearing area of the medial femoral condyle or from the intercondylar notch. Subsequently, with the second generation ACI techniques, a collagen membrane, as opposed to a periosteal patch, is used to cover the lesion. For the third generation ACI techniques, the cultivated chondrocytes are seeded into a three-dimensional biomaterial scaffold such as with HA, collagen, or an artificial scaffold a few days prior to implantation. Use of the artificial scaffold, refers to the third generation of chondrocyte transplantation techniques that is commonly called the MACI technique. As an illustration, these scaffolds can be protein based, carbohydrate based, or synthetic polymers, which can be woven, spun into nanofibers, or configured as hydrogels. By the time the scaffolds are loaded with cells, bioreactors are used to provide nutrients and mechanical stimulation to further develop the cultured chondrocytes. In essence, these biocompatible scaffolds allow a more efficient execution of implanting cultured chondrocytes, without the need of a knee arthrotomy, as the use of a matrix no longer requires injection of cells directly into the lesion. Although these later techniques of ACI can be performed all arthroscopically, some locations of defects, such as on the patella, still require an arthrotomy to be implemented for accessibility. After preparing the recipient bed and prior to implantation of the scaffold, a mapper or sizer is used to determine the shape and size of the cartilage defect. As soon as measurements are obtained, the cultured cartilage scaffold is trimmed and tailored to the appropriate size, then implanted into the defect, after removal of solution from the joint, and fixed with fibrin glue. Important to realize, while implanting the scaffold, the cell-seeded side of the implant needs to be placed facing down on the subchondral bone. Under these circumstances, given the efficiency of the subsequent ACI techniques, additional and concurrent interventions can be performed during the same surgical setting.
Postprocedure Healing and Rehabilitation
The postoperative healing, after an ACI technique, proceeds through three phases—proliferation, transition, and remodeling. For this reason, rehabilitation following ACI corresponds with the distinct phases of healing. In the first 6 weeks post procedure, the implanted chondrocytes are undergoing the proliferation phase. To begin with, over 12–18 hours after implantation, cells are allowed to adhere to the subchondral bone. To allow this cell adherence, knee motion is initially restricted for the first 18–24 h. After the short immobilization period, continuous passive motion (CPM) is begun to provide a chondrogenic stimulus. The importance behind use of continuous passive motion has been emphasized by studies showing the synthesis of glycosaminoglycans, chondroitin sulfate, and type II collagen. In addition, use of CPM stimulates the correct spatial distribution of chondrocytes. Along with passive mobilization of the knee, active mobilization of the ipsilateral hip and ankle, with concurrent isometric exercises, is conducted. During the proliferative phase, while soft primitive repair tissue is formed, no weight-bearing is allowed.
The second phase of cartilage healing, known as the transition phase, starts at postprocedure week 7 and lasts for 4–6 months. Depending on the size and location of the lesion treated with ACI, progressive weight-bearing is started with the goal of progressing to full weight-bearing. During this phase, exercises are aimed at achieving full range of motion while restoring full function of the muscles. However, open chain exercises are still avoided during this phase to prevent shear forces across the joint. Throughout this phase of healing, the chondrocytes release matrix into a puttylike consistency. Starting at 6 months and lasting for 12 months, cartilage healing proceeds through the remodeling phase. During this final phase, activities are resumed and progressively proceed toward regular activities by 1 year. At the final phase, the cartilage tissue actively hardens to firm cartilage similar to the adjacent native articular cartilage; yet, the graft continues to mature up to 3 years after ACI.
The gold standard for assessment, post ACI procedure, is arthroscopy. With arthroscopy, the repaired cartilage defect is able to be directly viewed, stiffness of the healed cartilage can be measured by probe indentation, and histomorphologic assessment can be conducted by obtaining a biopsy. Despite this gold standard, MRI is an alternative option that can provide viable information about the treated cartilage defect. In a study by Henderson et al., findings on MRI were able to be followed with second-look arthroscopy and histologic analysis of core biopsies. At 3 months after ACI, MRI demonstrated that 75% of defects had at least 50% defect fill along with 46% having a normal signal and 67% having no significant underlying bone marrow edema. At both 12 and 24 months, the demonstrations on MRI continued to improve to above 95% with defect fill and normal cartilage signal. PostACI assessment continues to be further investigated with studies searching for correlations with functional outcomes in both short-term and long-term follow-up.
Applications of Autologous Chondrocyte Implantation
One of the first studies to evaluate the ACI technique in the United States was performed by Micheli and colleagues on 50 patients in 19 centers across the country. In this study, the mean defect size was 4.2 cm 2 and 39 of the 50 patients had a prior articular cartilage procedure performed in the knee before receiving an ACI. Regardless of the previous treatment, or the size of the defect, there was no ramification on the results after ACI. At 36 months after ACI, 94% of the patients healed without failure of the graft. Intermediate results, at 2–9 years of follow-up, after ACI were reported on 101 patients. From this cohort, results correlated with location of lesion with good to excellent results in 92% with an isolated femoral condyle lesion, 67% with multiple lesions, 89% with osteochondritis dissecans, and 65% with a patella lesion. In the final analysis, a learning curve for the ACI procedure was demonstrated by four failures that occurred in the first 23 patients but only three failures that occurred in the last 78 patients. Long-term results, at 5–11 years of follow-up, were reported on 61 patients post ACI procedure. After follow-up of 5–11 years, 51 of the 61 patients had good to excellent results. At the same time, second-look reevaluations of 8 out of the 12 biopsies showed hyaline-like repair tissue at the grafted ACI site. Given these points, a durable outcome, for as long as 11 years after ACI, was reported consistently throughout the years of follow-up in the cohort. From multiple studies, key prognostic factors have been determined to aid in selecting the appropriate candidates for intervention with an ACI technique. Compelling evidence has elucidated the following key factors for ACI: etiology, physiologic age, social factors, patient compliance, timed allowed for recovery, and desired postoperative activity level. With this in mind, complications rates, after ACI, have been reported to be higher with open procedures, with use of a periosteal graft cover, and with first generation ACI techniques. On the other hand, best outcomes after ACI, have been reported with younger patients, with shorter preoperative duration of symptoms, and with fewer prior surgical procedures. In detail, in a study by Knutsen et al., patients younger than 30 years, who underwent an ACI technique for treatment of a cartilage defect, exhibited significantly better Lysholm and Short-Form-36 (SF-36) scores at both 2 and 5 years in comparison to patients older than 30 years who received the same treatment. All things considered, studies on ACI support improved outcomes in the majority of patients and optimal outcomes in patients with positive prognostic factors.
With the succession of different techniques for ACI, studies have been conducted to clarify the clinical outcomes between procedures. In comparison between first and second generation ACI techniques, studies have shown equivalent short-term clinical outcomes as well as similar complications and reoperation rates. In a randomized study by Bartlett et al., 91 patients were randomized to receive either a first generation ACI technique with a type I/type III collagen cover or to receive a third generation ACI technique with a collagen bilayer seeded with chondrocytes. From this study both generations of ACI resulted in clinical improvement at 1 year. After 1 year, arthroscopic assessment and biopsies showed hyaline cartilage in 43.9% of first generation ACI and 36.4% of third generation ACI; however, there was no difference in reoperation with both groups undergoing additional procedures at 9%. At short-term follow-up, between both generations of ACI, there was no noted significant difference in clinical, arthroscopic, or histologic outcomes. In another randomized study by Zeifang et al., 21 patients with full-thickness cartilage defects at the femoral condyle were randomized to undergo either matrix-associated autologous chondrocyte implantation or the original periosteal flap ACI technique. At both 1 and 2 years of follow-up, there was no significant difference in knee function as assessed by the International Knee Documentation Committee (IKDC) score between the different generations of ACI techniques. In addition, there were no major differences in complications between the treatment groups. As can be seen, short-term results show no significant differences between the different generations of ACI techniques, yet further studies, with longer follow-up, are required to determine the durability between the various techniques of chondrocyte implantation.
The initial ACI technique required an arthrotomy to provide access for injection of the cultured chondrocytes while subsequent ACI techniques are amenable to all arthroscopic procedures by use of a scaffold; given these circumstances, the difference in outcome between open and arthroscopic ACI techniques have been evaluated in several studies. In a systematic review by Harris et al., comparison of open versus arthroscopic ACI resulted in more rapid improvement, fewer complications, and a lower rate of reoperation with the all arthroscopic ACI technique. In a prospective study comparing open and arthroscopic ACI techniques, at 5 years follow-up, results demonstrated significantly improved and better functional outcomes in favor of the all arthroscopic group. Not to mention, up to 19 months after surgery, the arthroscopic ACI group improved and remained stable up to the 5-year follow-up mark, while the open ACI group continued to improve up to 24 months after surgery. Other options to treat cartilage defects, particularly small contained defects, include microfracture or osteochondral grafts, and several studies have compared these techniques to ACI. Important to realize, the summation of studies comparing ACI techniques to microfracture techniques determine better short- and intermediate-term clinical results with ACI implantation. Overtime, clinical outcomes following microfracture tend to plateau or even decline at longer periods of follow-up. In contradistinction, clinical outcomes following ACI techniques tend to remain stable or even continue to improve at longer periods of follow-up. In like matter, in a randomized study by Saris et al., 108 patients with single ICRS grade III/IV cartilage defects of the femoral condyle were randomized to receive microfracture or chondrocyte implantation. At 36 months follow-up, the chondrocyte implantation group resulted in significantly better clinical outcomes while the microfracture group resulted in decline in clinical outcomes. With this in mind, the promising results with ACI techniques led into the evaluation of the cost-effectiveness for treatment of cartilage lesions. Minas and colleagues evaluated the quality of life of 44 patients who had undergone an ACI procedure and calculated the average cost per additional quality-adjusted life year. Up to 24 months post ACI, functional improvements were noted in multiple outcome scores as well as quality of life, as measured by the Short-Form-36 Score. After analysis, the estimated cost per additional quality-adjusted life year was $6701. For these reasons, ACI was determined to be a cost-effective treatment for cartilage lesions of the knee.
Safety of Autologous Chondrocyte Implantation
Given the widespread use of ACI, reports of complications have been collected overtime. With the use of living cultured chondrocytes, one concern is the development of graft hypertrophy. In a systematic review by Harris et al., graft hypertrophy has been noted in 22% after periosteal ACI, 6% after collagen membrane ACI, 4% after Hyalograft C ACI, and 7% after MACI. Arthrofibrosis, especially following open ACI techniques, has been noted to occur in about 2.5% of ACI techniques. After a review of 604 cases, following an ACI procedure, failure or reoperation occurred in 2.8% (17) cases. Important to realize, the rate of failure was noted to increase threefold if a microfracture procedure was performed prior to an ACI technique. Less common complications, following an ACI technique, after review of 13 studies including 917 subjects were reported as three superficial wound infections, one septic arthritis, two reflex sympathetic dystrophies, and two deep venous thromboses. All things considered, the lower rate of complications and lower rate of reoperations have been noted with the all arthroscopic ACI techniques.
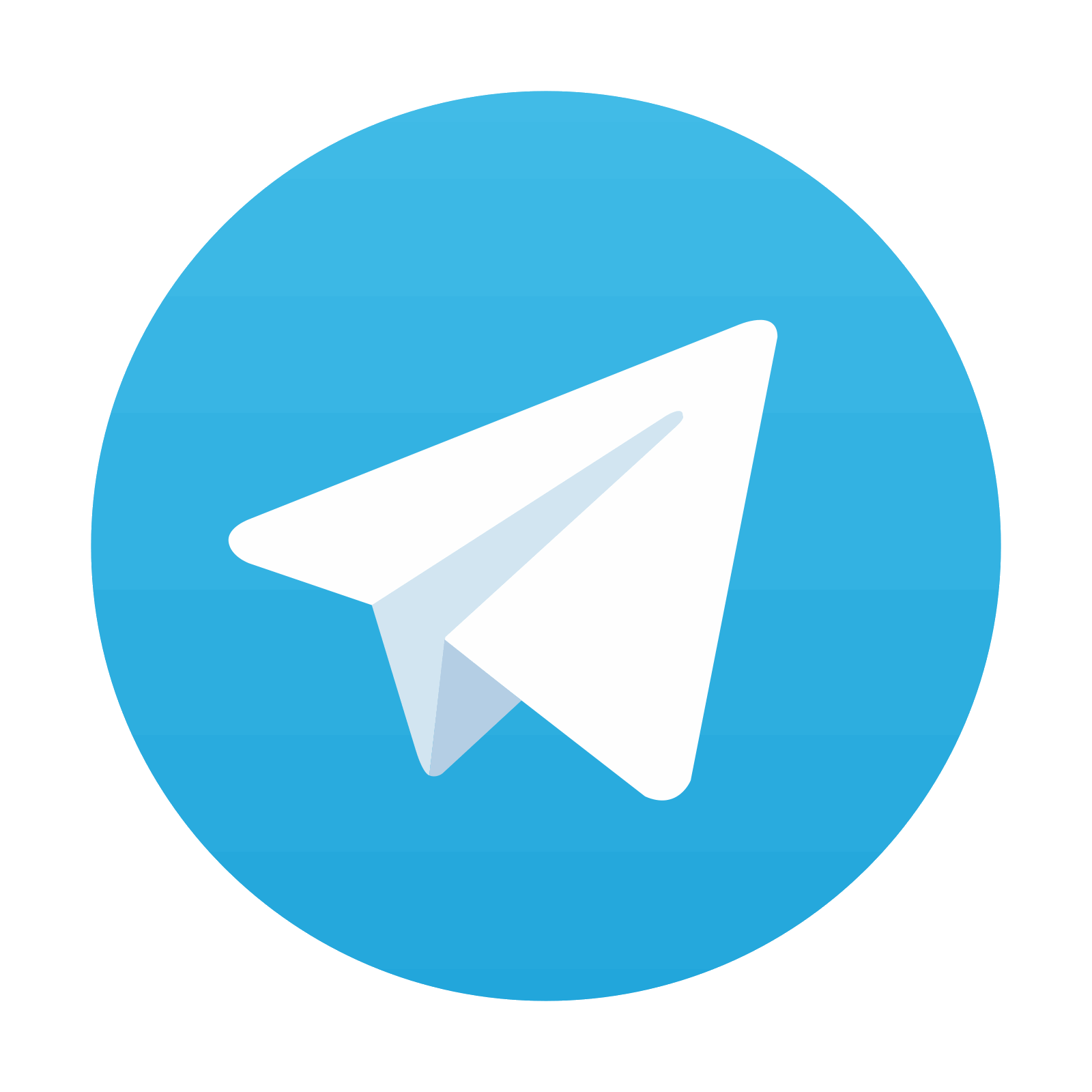
Stay updated, free articles. Join our Telegram channel
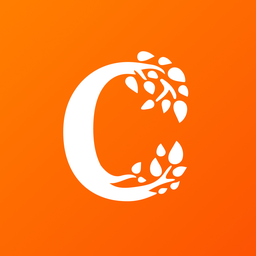
Full access? Get Clinical Tree
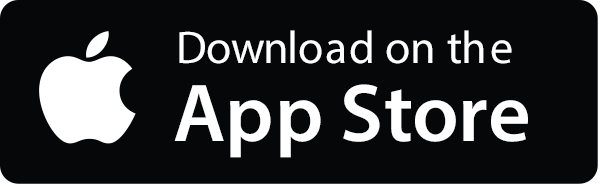
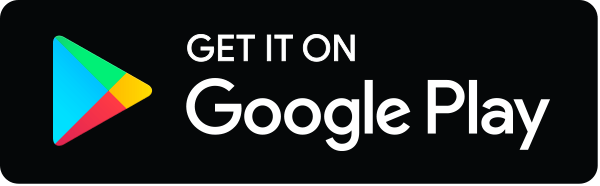