Abstract
Non-invasive imaging has great potential to contribute to the ‘Three R’s’ principles for more ethical use of experimental animals. It enables repetitive monitoring of disease progression and measurement of quantitative biomarkers that report on disease progression and therapy efficacy in the same animal, thereby reducing manifold the number of animals needed for in vivo studies whilst advancing our knowledge into the pathophysiology of these diseases.
This article reviews applications of non-invasive imaging in the field of fibrosis and arthritis research. It provides evidence for the viability of this approach not only for ethical reasons (reducing numbers and suffering in research animals, according to the 3R principles) but also for accelerating experimental output and making it more translational. The emphasis is on promising developments which will help improving throughput by reducing experiment length and size as well as human resources for data analysis, therefore encouraging a wider spreading of novel imaging technologies.
Introduction
Studies on human conditions involving a complex interplay of environmental and host factors such as in the Rheumatology field cannot but rely on animal models with a genetic or induced disease that is similar to the human disorder. Therefore, simply avoiding animal testing is not always feasible in order to achieve the same scientific aims. Considering the guiding principles of the ‘Three R’s’ for more ethical use of animals in laboratory practice that were first described by Russell and Burch in 1959, there is more to it than simply feeling encouraged to avoid animal experimentation by ‘replacement’ . ‘Reduction’ using tools that enable researchers to obtain more information from fewer animals and ‘Refinement’ using methods that avoid, alleviate, or minimize any potential suffering, pain, or distress of the animals are at least as important . Small animal models that mimic important aspects of rheumatic disease, such as fibrosis and arthritis, are widely used for target discovery and drug development in the field.
Semi-quantitative assessment by histological analysis of isolated organs post mortem is the current gold standard, but requires many animals to be killed at several time points during the study. While these ex vivo analyses on tissue samples can provide the most detailed cellular and molecular analysis of the disease, they are intrinsically unable to follow the kinetics of disease and host response processes which are, by definition, dynamic in space and time. Ex vivo analyses also carry the ethical burden of requiring the use of many animals to overcome the statistical issues inherent to high inter-animal variation that would otherwise reduce the power of experiments.
Two-dimensional (2D) sampling is too limited for processes that are not evenly distributed throughout an entire organ such as the pulmonary lobes in lung fibrosis. Isolation of the lung for ex vivo assessments also suffers from some specific issues such as distortion of lung structure during fixation, thus resulting in potential artefacts and biased alveolar properties. Because non-invasive pulmonary function measurements have limitations regarding sensitivity, specificity and available readout , current developments in nondestructive imaging, with the ability to assess the disease in situ, hold great promise in this regard. Similarly, the volumetric reconstruction of the joint morphology obtained with non-destructive imaging offers a superior localisation of arthritic lesions, increased precision in the assessment of the severity and the possibility of modelling joint mechanics.
However, the spatial localisation of morphological and molecular alterations achieved through non-destructive/in situ imaging becomes truly informative when such changes can be determined longitudinally in the same individual, which provides the otherwise missing knowledge about their temporal progression and interaction. There is therefore a strong requirement for tools that enable the repeated measurement of dynamic processes in the same animal in vivo and that are therefore non-invasive.
Home Office statistics on the use of research animals in the UK show that mice are by far the most frequently used species and the number of mice used between 2004 and 2014 has increased, while for larger rodents such as rats, this trend was opposite . Due to the amenability of mice to genetic modifications, which allows to testing specific molecular hypothesis in vivo, their use in experimental labs is predicted to further increase in future years. This suggests that, in order to achieve a significant reduction in the use of experimental animals, non-invasive imaging technologies will have to be optimised for the mouse.
The aim of this review is to describe the available non-invasive imaging technologies for the assessment of arthritis and fibrosis in small rodent models of these pathologies, with a focus on new promising developments in this area and on the impact of present and future imaging technologies on the 3Rs principles.
Animal models
Animal models used in the field of systemic rheumatic diseases always highlight certain aspects of rheumatology or systemic syndromes and will, as such, focus on an important manifestation of the disease. There is no true experimental model that exactly reproduces human disease. Animal models are induced to reproduce the pathological conditions of the patients as faithfully as possible, but, as the aetiology of many rheumatic conditions is still unclear, one has to rely on the induction of certain known aspects of a disease. Despite their imperfections, animal models are indispensable for research and many insights we know today that we have gained through help from our little friends.
Animal models of arthritis
Animal models are essential for the research on rheumatoid arthritis (RA) and osteoarthritis (OA) due to the incomplete understanding of the aetiology and mechanisms of these diseases. This causes that the entire organisms needs to be assessed in order to avoid missing potentially significant, yet unknown, systemic or local contributions. In this context, murine models are particularly attractive because of their amenability to genetic alterations which allow studying the role of specific molecular patterns in the disease mechanisms. The murine models of RA and OA currently in use can be divided in two main categories based on the origin of the pathology: 1) the models where the disease is naturally occurring (e.g. with ageing certain strains) or genetically induced (through artificial genomic alterations) and 2) the models where the pathology is secondary to an external intervention (e.g. intra-articular chemical injection or joint destabilising surgery to induce OA and immune sensitisation to collagen type II to generate RA). The main advantage of the models induced by external intervention is that the exact point of initiation of the pathology is known, which simplifies disease monitoring and staging across uniform samples. Genetically modified mice have been increasingly used in physically induced disease models to assess the role of molecular pathways in accelerating pathology or protecting from it . This article does not describe in detail all different small rodent models of RA and OA and interested readers are referred to dedicated reviews .
From an imaging point of view, all these different animal models hold similar requirements in terms of the morphological and functional assessment of the joint pathology. Specific pathological features of RA and OA are described in greater detail later in relation to their respective imaging methods.
Animal models of (lung) fibrosis
Systemic sclerosis is an autoimmune disease that is currently incurable. Its aetiology remains unknown. It manifests as scleroderma and visceral organ involvement: fibrosis would occur in the gastrointestinal tract and the heart and, importantly, in the lungs . Pulmonary fibrosis is a frequent and an important complication of systemic sclerosis. It has a poor prognosis and not much is known about the molecular and cellular events that underlie the development of lung fibrosis in systemic sclerosis. Only in vivo models can try to reproduce the complex interplay of genetic and host immune factors and biochemical and tissue interactions that combine to cause lung fibrosis. Several animal models of dermal fibrosis and systemic sclerosis exist that aid researchers to unravel these complex events . The currently used small animal models of lung fibrosis and other common human lung diseases remain limited in terms of their ability to truly recapitulate human pathophysiologic conditions. The ideal animal model for systemic sclerosis would recapitulate all aspects of widespread vasculopathy, autoimmunity with production of antibodies and progressive fibrosis. Yet, no animal model that would phenocopy all hallmarks of the disease is available; however, several models that enable the study of a certain aspect related to vasculopathy, inflammation, autoimmunity or fibrosis are available. These animal models can be divided into inducible and genetic models.
Animal models to replicate human fibrosis are often fabricated by inducing a fibrotic response upon the introduction of a pro-fibrotic agent or thoracic irradiation. For studying the processes of both pulmonary and skin fibrosis, the bleomycin-induced rodent model is by far the most widely used and best characterized model. Bleomycin is an antibiotic that was used in oncology because of its antineoplastic properties, but this use was limited by dose-dependent toxicity manifesting as ‘pulmonary fibrosis’. It could, however, be used to induce and model fibrosis in a wide variety of animals (dogs, primates and rodents), over a wide range of doses and via different administration routes (intraperitoneal, intratracheal, intravenous or subcutaneous) . Bleomycin causes oxidative stress and DNA strand breakage in organs that are sensitive due to the insufficient presence of bleomycin hydrolase, such as the lungs. Single intra-tracheal bleomycin injection results in an acute lung injury with epithelial damage and capillary leaks, thus resulting in alveolitis via upregulation of pro-inflammatory cytokines and chemokines (tumour necrosis factor (TNF)α, interleukin (IL)-1 and IL-6) . Transforming growth factor (TGF)-β is a crucial mediator . During the second week after induction, transition from inflammation to fibrosis is observed, established by fibroblast proliferation, expansion of myofibroblasts, extracellular matrix deposition and abundance of pro-fibrotic cytokines, with maximal fibrosis observed after 3–4 weeks. Beyond the fourth week after induction, fibrotic outcomes are increasingly variable through the initiation of processes that may resolve fibrosis .
It is well known that this model does not reflect all aspects of human lung fibrosis; nonetheless, it has been extensively used during the last decades. The lack of progressive fibroproliferation and even spontaneous recovery is a major issue with this model as one for human fibrotic pathology that is always progressive in nature, for both idiopathic pulmonary fibrosis as systemic sclerosis-related interstitial lung disease . The clear TGFβ signature gives it nevertheless important relevance to study signalling pathways during fibrosis processes. But when evaluating this model for drug development, the translation of preclinical compounds into the clinic has remained disappointing . This questions the relevance of this model for any other lung fibrotic condition than bleomycin-induced lung fibrosis.
In spite of its shortcomings, the bleomycin-induced model could still be a relevant model for truly anti-fibrotic drug development, given that drugs are tested not in a prophylactic but truly therapeutic manner. The course of disease in this mouse model is very short compared to human disease and has a strong inflammatory component, whereas in this phase, the human disease remains often unrecognized. This implies that it is crucial to start therapy only during the fibrotic phase of the disease, implying the challenging identification of the time of shift from mainly inflammatory to fibrotic processes for each animal individually. Given the short time window that this model provides, this is very challenging. The FITC model shows persistent fibrosis up to months which facilitates antifibrotic therapy testing, but is not clinically relevant . Better models have been developed that involve repeated bleomycin administration. Degryse et al. have published refinements of the bleomycin model that do perform better in terms of recapitulating fibroblastic foci, albeit rarely, that are believed to better represent the deregulation of processes in the lung that underlie idiopathic lung fibrosis . More people have published refinements of more traditional animal models that have given many insights into pathophysiology (reviewed in Ref. ).
Genetic models of lung fibrosis, including transgenic and locally transgenic animals, are useful because they offer insights into pathogenesis of fibrosis by known causes. A locally transgenic model which caused the appearance of abundant fibroblastic foci in the lung with progressive fibrosis over 1–2 months after the challenge made use of the intratracheal injection of engineered viral vectors to topically introduce a profibrotic gene, transforming growth factor TGF-β1 . Adenoviral-mediated delivery of granulocyte macrophage–colony-stimulating factor, TNF and IL-1 all produce variable degrees of pulmonary fibrosis that share morphologic similarities with that induced by pulmonary TGF-β1 overexpression . Transgenic animals used in the context of lung fibrosis research mostly have genetic defects that manipulate their susceptibility to induce fibrosis, for example, overexpression of certain cytokines, fibrinolytic pathway proteins, extracellular matrix components or tissue proteinases. Genetic models are also available, such as the tight-skin1 (Tsk1) mice that are heterozygous for a mutation in the fibrillin1 gene which causes them to develop skin and lung fibrosis. In contrast to human dermal fibrosis, the thickened skin in Tsk1-mice results from a thickened hypodermis . Fos-related antigen-2 (Fra2) is a transcription factor involved in proliferation, apoptosis, fibrosis and tumourigenesis. A Fra2-transgenic mouse has peripheral microvasculopathy with decreased capillary density, perivascular inflammatory infiltrates and apoptosis of dermal endothelial cells, preceding the development of skin fibrosis. In the lungs, Fra2-mice develop pulmonary vasculopathy that evolves into pulmonary fibrosis. The presence of both peripheral and pulmonary vasculopathy that precedes fibrosis resembles many aspects of human systemic sclerosis . All experimental models of fibrosis suffer from the fact that animal models attempt to reproduce in a very short period of time a disease process that happens in humans over many months and years of aberrant matrix remodelling. Assessing preclinical models with techniques that only provide a single measurement of dynamic processes narrows even more this small time window down to a snapshot that is difficult to interpret in the context of a progressing disease. While in animal models, fibrosis is often the result of a certain induced event, it becomes only more relevant to try to monitor longitudinally the events during disease progression in animals, before extrapolating these data to the time course of what may happen during human disease.
Animal models
Animal models used in the field of systemic rheumatic diseases always highlight certain aspects of rheumatology or systemic syndromes and will, as such, focus on an important manifestation of the disease. There is no true experimental model that exactly reproduces human disease. Animal models are induced to reproduce the pathological conditions of the patients as faithfully as possible, but, as the aetiology of many rheumatic conditions is still unclear, one has to rely on the induction of certain known aspects of a disease. Despite their imperfections, animal models are indispensable for research and many insights we know today that we have gained through help from our little friends.
Animal models of arthritis
Animal models are essential for the research on rheumatoid arthritis (RA) and osteoarthritis (OA) due to the incomplete understanding of the aetiology and mechanisms of these diseases. This causes that the entire organisms needs to be assessed in order to avoid missing potentially significant, yet unknown, systemic or local contributions. In this context, murine models are particularly attractive because of their amenability to genetic alterations which allow studying the role of specific molecular patterns in the disease mechanisms. The murine models of RA and OA currently in use can be divided in two main categories based on the origin of the pathology: 1) the models where the disease is naturally occurring (e.g. with ageing certain strains) or genetically induced (through artificial genomic alterations) and 2) the models where the pathology is secondary to an external intervention (e.g. intra-articular chemical injection or joint destabilising surgery to induce OA and immune sensitisation to collagen type II to generate RA). The main advantage of the models induced by external intervention is that the exact point of initiation of the pathology is known, which simplifies disease monitoring and staging across uniform samples. Genetically modified mice have been increasingly used in physically induced disease models to assess the role of molecular pathways in accelerating pathology or protecting from it . This article does not describe in detail all different small rodent models of RA and OA and interested readers are referred to dedicated reviews .
From an imaging point of view, all these different animal models hold similar requirements in terms of the morphological and functional assessment of the joint pathology. Specific pathological features of RA and OA are described in greater detail later in relation to their respective imaging methods.
Animal models of (lung) fibrosis
Systemic sclerosis is an autoimmune disease that is currently incurable. Its aetiology remains unknown. It manifests as scleroderma and visceral organ involvement: fibrosis would occur in the gastrointestinal tract and the heart and, importantly, in the lungs . Pulmonary fibrosis is a frequent and an important complication of systemic sclerosis. It has a poor prognosis and not much is known about the molecular and cellular events that underlie the development of lung fibrosis in systemic sclerosis. Only in vivo models can try to reproduce the complex interplay of genetic and host immune factors and biochemical and tissue interactions that combine to cause lung fibrosis. Several animal models of dermal fibrosis and systemic sclerosis exist that aid researchers to unravel these complex events . The currently used small animal models of lung fibrosis and other common human lung diseases remain limited in terms of their ability to truly recapitulate human pathophysiologic conditions. The ideal animal model for systemic sclerosis would recapitulate all aspects of widespread vasculopathy, autoimmunity with production of antibodies and progressive fibrosis. Yet, no animal model that would phenocopy all hallmarks of the disease is available; however, several models that enable the study of a certain aspect related to vasculopathy, inflammation, autoimmunity or fibrosis are available. These animal models can be divided into inducible and genetic models.
Animal models to replicate human fibrosis are often fabricated by inducing a fibrotic response upon the introduction of a pro-fibrotic agent or thoracic irradiation. For studying the processes of both pulmonary and skin fibrosis, the bleomycin-induced rodent model is by far the most widely used and best characterized model. Bleomycin is an antibiotic that was used in oncology because of its antineoplastic properties, but this use was limited by dose-dependent toxicity manifesting as ‘pulmonary fibrosis’. It could, however, be used to induce and model fibrosis in a wide variety of animals (dogs, primates and rodents), over a wide range of doses and via different administration routes (intraperitoneal, intratracheal, intravenous or subcutaneous) . Bleomycin causes oxidative stress and DNA strand breakage in organs that are sensitive due to the insufficient presence of bleomycin hydrolase, such as the lungs. Single intra-tracheal bleomycin injection results in an acute lung injury with epithelial damage and capillary leaks, thus resulting in alveolitis via upregulation of pro-inflammatory cytokines and chemokines (tumour necrosis factor (TNF)α, interleukin (IL)-1 and IL-6) . Transforming growth factor (TGF)-β is a crucial mediator . During the second week after induction, transition from inflammation to fibrosis is observed, established by fibroblast proliferation, expansion of myofibroblasts, extracellular matrix deposition and abundance of pro-fibrotic cytokines, with maximal fibrosis observed after 3–4 weeks. Beyond the fourth week after induction, fibrotic outcomes are increasingly variable through the initiation of processes that may resolve fibrosis .
It is well known that this model does not reflect all aspects of human lung fibrosis; nonetheless, it has been extensively used during the last decades. The lack of progressive fibroproliferation and even spontaneous recovery is a major issue with this model as one for human fibrotic pathology that is always progressive in nature, for both idiopathic pulmonary fibrosis as systemic sclerosis-related interstitial lung disease . The clear TGFβ signature gives it nevertheless important relevance to study signalling pathways during fibrosis processes. But when evaluating this model for drug development, the translation of preclinical compounds into the clinic has remained disappointing . This questions the relevance of this model for any other lung fibrotic condition than bleomycin-induced lung fibrosis.
In spite of its shortcomings, the bleomycin-induced model could still be a relevant model for truly anti-fibrotic drug development, given that drugs are tested not in a prophylactic but truly therapeutic manner. The course of disease in this mouse model is very short compared to human disease and has a strong inflammatory component, whereas in this phase, the human disease remains often unrecognized. This implies that it is crucial to start therapy only during the fibrotic phase of the disease, implying the challenging identification of the time of shift from mainly inflammatory to fibrotic processes for each animal individually. Given the short time window that this model provides, this is very challenging. The FITC model shows persistent fibrosis up to months which facilitates antifibrotic therapy testing, but is not clinically relevant . Better models have been developed that involve repeated bleomycin administration. Degryse et al. have published refinements of the bleomycin model that do perform better in terms of recapitulating fibroblastic foci, albeit rarely, that are believed to better represent the deregulation of processes in the lung that underlie idiopathic lung fibrosis . More people have published refinements of more traditional animal models that have given many insights into pathophysiology (reviewed in Ref. ).
Genetic models of lung fibrosis, including transgenic and locally transgenic animals, are useful because they offer insights into pathogenesis of fibrosis by known causes. A locally transgenic model which caused the appearance of abundant fibroblastic foci in the lung with progressive fibrosis over 1–2 months after the challenge made use of the intratracheal injection of engineered viral vectors to topically introduce a profibrotic gene, transforming growth factor TGF-β1 . Adenoviral-mediated delivery of granulocyte macrophage–colony-stimulating factor, TNF and IL-1 all produce variable degrees of pulmonary fibrosis that share morphologic similarities with that induced by pulmonary TGF-β1 overexpression . Transgenic animals used in the context of lung fibrosis research mostly have genetic defects that manipulate their susceptibility to induce fibrosis, for example, overexpression of certain cytokines, fibrinolytic pathway proteins, extracellular matrix components or tissue proteinases. Genetic models are also available, such as the tight-skin1 (Tsk1) mice that are heterozygous for a mutation in the fibrillin1 gene which causes them to develop skin and lung fibrosis. In contrast to human dermal fibrosis, the thickened skin in Tsk1-mice results from a thickened hypodermis . Fos-related antigen-2 (Fra2) is a transcription factor involved in proliferation, apoptosis, fibrosis and tumourigenesis. A Fra2-transgenic mouse has peripheral microvasculopathy with decreased capillary density, perivascular inflammatory infiltrates and apoptosis of dermal endothelial cells, preceding the development of skin fibrosis. In the lungs, Fra2-mice develop pulmonary vasculopathy that evolves into pulmonary fibrosis. The presence of both peripheral and pulmonary vasculopathy that precedes fibrosis resembles many aspects of human systemic sclerosis . All experimental models of fibrosis suffer from the fact that animal models attempt to reproduce in a very short period of time a disease process that happens in humans over many months and years of aberrant matrix remodelling. Assessing preclinical models with techniques that only provide a single measurement of dynamic processes narrows even more this small time window down to a snapshot that is difficult to interpret in the context of a progressing disease. While in animal models, fibrosis is often the result of a certain induced event, it becomes only more relevant to try to monitor longitudinally the events during disease progression in animals, before extrapolating these data to the time course of what may happen during human disease.
Non-invasive and non-destructive visualisation of lung fibrosis
It is impossible to imagine clinical practice nowadays without imaging technology that provides doctors with the opportunity to view diseased lungs through a computed tomography (CT) scan of their patients’ chests. This imaging technology would have also enormous benefits for the monitoring of dynamic changes regarding lung disease in live animals in a research context. Nevertheless, its extension to animals is not that obvious. It is particularly challenging to image lungs, especially in small animals such as mice. As you cannot ask a mouse to hold its breath, the motion caused by its fast respiration poses a technological challenge to reach the high resolution and sensitivity required for obtaining meaningful images from an animal that is about a thousand times smaller than a human. Notwithstanding the challenge, investigators and engineers are working together to make imaging technology available and better for use with small laboratory animals because of the huge benefits it has for preclinical research. Despite the efforts in exploring different technologies for preclinical lung imaging (reviewed in Ref. ), the application of imaging techniques for the specific assessment of lung fibrosis in vivo is more limited.
Micro-computed tomography (micro-CT) offers high-resolution images of the lungs in a preclinical context, making use of the natural inherent contrast of air versus lung tissue and dense pathology such as fibrotic lesions. In recent years, micro-CT has proven to be an excellent tool for monitoring lung disease and therapy repeatedly in a single animal ( Fig. 1 ), which is a huge advantage. Several studies have provided proof-of-principle studies of the feasibility of using micro-CT for the visualization of lung fibrosis, thus leading to the demonstration that high-resolution micro-CT is capable of providing longitudinal images of a quality high enough to allow automated quantification of the bleomycin-induced fibrosis process, while being safe for the animal under investigation .
For dealing with respiratory motion, most micro-CT scanners can be connected to an animal ventilator that triggers the scanner to acquire images during set phases of the respiratory cycle, known as prospective respiratory gating. Most imaging is done during the relatively motion-free expiration phase, but better contrast is achieved when the lungs contain more air during inspiration. This requires intubation of the animal and inversion of the breathing cycle and careful interpretation of the physiological relevance of the readout. Another option is to perform micro-CT of free-breathing animals, such that lung volumes extracted from the four-dimensional (4D) CT data would reflect truly physiological conditions and therefore vital parameters of the animals. A high-resolution respiratory-gated 4D micro-CT protocol with a nevertheless short scanning time could be repeatedly used in free-breathing mice with zero mortality . Such a non-invasive approach is highly relevant when working with lung disease models, as these animals would suffer from the repeated intubations applied for breath-hold-gated or prospectively gated micro-CT protocols .
However, one hurdle remains before micro-CT could be embraced by the lung fibrosis research community. Because of the higher resolution and sensitivity required to provide a good-quality mouse lung CT scan, the X-ray dose would also be higher. Knowing that lungs are particularly sensitive for side effects from radiation, any concerns regarding the possible induction of lung fibrosis because of the X-ray exposure during scanning must be excluded before using micro-CT for animal studies. Fortunately, the radiation dose that a mouse is exposed to during a lung micro-CT scan turned out to be much lower than doses used for cancer radiotherapy that are known to cause side effects . Any potentially harmful effects when repeatedly scanning the same mouse could be excluded, thereby demonstrating the safety of micro-CT. This was done for different scanning protocols that involve scans once or twice a week up to 3 months in a row, long enough to cover a typical preclinical study with induced or transgenic animal models.
Notwithstanding that, it is important to keep in mind that an effect of repeated radiation exposure on the disease process itself cannot be excluded, but appears rather unlikely or very limited compared to the disease-related changes that are observed. While the relatively low cumulative radiation dose of weekly repeated micro-CT scans falls well within a healthy mouse’s repair capacity , it is more difficult to evaluate the possible effects of X-rays on the inflammatory and tissue remodelling processes specific to lung fibrosis models. In those cases, recent advances in lung ‘magnetic resonance imaging (MRI)’ may provide an alternative that is devoid of any concerns regarding radiotoxic influences on the disease process.
MRI of lung is particularly difficult. It is mainly filled with air such that its proton density is low, which results in a very low inherent signal available for imaging. Moreover, the many tissue–air interfaces form very different magnetic environments, thus resulting in magnetic susceptibility effects that cause imaging artefacts and accelerate signal decay of the already low lung MR signal. As a consequence, with the parameters (echo times) used in conventional MRI protocols, little or no signal is left to image so that lung tissue appears dark on MR images. Nonetheless, the zero signal in the lungs provides a useful background against which abnormalities that cause an increase in signal, such as lung fibrosis or cellular infiltration, are recognized . In fact, the feasibility of a conventional lung MRI approach for the visualization of bleomycin-induced disease processes in the lung has been demonstrated in a rat model and in mice . Yet, these susceptibility effects at tissue/air interfaces may hinder the early detection of smaller fibrotic patches or sites of disease onset at earlier stages of pathogenesis when using conventional MRI protocols. To overcome this, a pulse sequence allowing for ultra-short echo times (UTE) has been developed . In UTE MRI, image contrast is much less influenced by air-tissue susceptibility effects and the detectability of tissues that particularly suffer from these effects, such as lung tissue and fibrotic tissue, has therefore improved significantly. UTE MRI is currently under evaluation for clinical applications , but was only recently introduced for mouse lung imaging . Its ability to sensitively evaluate lung fibrosis development and therapy evaluation in the bleomycin-induced model has been recently shown ( Fig. 2 ) .
In addition to conventional and UTE MRI that detect structural changes in the lung, the use of hyperpolarized gases in combination with MRI helps evaluate lung function during the examination. This has been shown through the detection of inhaled 129 Xe or 3 He in the airspaces, lung interstitium, and red blood cells, thus showing impaired gas transfer in rats suffering from bleomycin-induced lung injury . The usefulness of this technique for routine small animal work is, however, limited, because accessibility, cost and complexity of this technique are restrictive. Due to technological advances, MRI has evolved from a modality with little utility for lung imaging to one having remarkable potential. Moreover, MRI is a much more versatile tool that enables monitoring of different parameters and contrast mechanisms, potentially providing more information on different pathogenic processes in the lung, ultimately aiming at discriminating between inflammatory and fibrotic processes in vivo. Although some evidence is available that MRI is indeed capable to provide a differential readout for inflammation versus fibrosis, it is currently not possible to reliably identify factors that distinguish between inflammatory versus fibrotic disease . This is nevertheless a relevant research challenge, motivated by its importance for the screening of potential anti-fibrotic as opposed to anti-inflammatory effects of experimental therapies, and justifying the use of the much more expensive MRI equipment.
Apart from using inherent tissue contrast such as in MRI and CT, a specific disease process can be demonstrated by labelling it with targeted contrast agents, such as with a genetic label or a molecular probe that binds to a cell or protein of interest. Radionuclide imaging techniques such as ‘positron emission tomography (PET)’ and ‘single photon emission computed tomography (SPECT)’ – with or without CT (or most recently, MRI) to provide the anatomical reference– have been explored for the imaging and quantification of fibrosis itself by targeting collagen or other components that are thought to be involved in pathogenic pathways in fibrosis development. As the hallmark of pulmonary fibrosis if the excessive production of extracellular matrix proteins, being mainly the proline-rich collagens, PET has been explored to detect the uptake of radiolabelled proline residues in experimental models of lung fibrosis. This, however, did not turn out to efficiently label the newly synthesized collagen in the extracellular matrix . Alternatively, 18 F-labelled glucose-analogue 18 F-FDG, the most widely used PET tracer, did not target collagen deposition, but sensitively detected the elevated cellular glucose uptake in rabbit models of lung inflammation and fibrosis and also in human patients . Its use is however limited because 18 F-FDG PET is highly unspecific and does not enable differential diagnosis with interstitial pneumonia. Moreover, 18 F-FDG has myocardial wall uptake which masks most of the pulmonary region in mice .
PET and SPECT have been used for the detection of specific molecular markers that are overexpressed during lung fibrosis and are thought to play a role in mediating the fibrotic response . Radionuclide imaging techniques in a clinical setting for the differential diagnosis of lung pathology is an active area of clinical imaging research due to their easy translatability and potential. Yet, neither PET nor SPECT has been used much for experimental pulmonary imaging. These modalities are somewhat less practical for routine use in a preclinical context, because of some limitations, such as its low sensitivity to small changes in signal strength and the potential substantial radiation dose associated with repeated examinations. Moreover, absolute quantification can be difficult as the radioisotope measured may not be the same as the imaging probe or target due to metabolism. PET also requires a nearby cyclotron and radiochemistry lab to create the isotopes and produce and purify the radiolabeled imaging probes and is relatively expensive. SPECT has some advantages such as the ability of sensing multiple probes and working with longer half-life isotopes and kit chemistry for simple probe labelling, but nevertheless has not been used extensively for preclinical fibrosis research.
Conversely, optical imaging is one of the most used in vivo imaging modalities in a preclinical context, because it is simple to understand, easy to use, and cheap. Whole-body optical imaging is of two types: ‘bioluminescence imaging (BLI)’, based on the production of photons through a light-producing chemical reaction, catalysed by a luciferase upon the administration of a substrate and ‘fluorescence imaging (FI)’, using excitation light to induce fluorescence from a fluorescent reporter protein or dye. FI suffers from tissue autofluorescence, which can be overcome in some cases using more complicated spectral imaging and unmixing algorithms. BLI would be more sensitive as there is virtually no background, but it requires genetic labelling of the target cells and is dependent on substrate administration kinetics and cofactor availability. An example could be quantifying the bioluminescence emitted through luciferase expression driven by a promoter that drives the expression of a key gene in fibrosis pathogenesis. Near-infrared fluorescent probes have been developed and used to detect matrix metalloproteinase (MMP) activity, which is thought to be involved in idiopathic lung fibrosis, although MMPs are not specific for fibrosis as they are also important in lung inflammation . Despite its popularity in preclinical research in general, optical imaging is hardly used in the lung and for lung fibrosis specifically . This might be due to some technical challenges associated with optical imaging in the lung. The scattering of light travelling through tissue is 2–3 times higher in the lung than in other nearby tissues such as heart and liver , because of the strong optical heterogeneity inherent to the lung microstructure . Improvements in optical imaging techniques will probably drive the development of improved molecular probes and vice versa. Developing probes for endogenous targets relevant in pulmonary fibrosis is relevant mainly in the light of preclinical investigations and even clinical translatability for detection with optical endoscopy, for example, but may turn out to be challenging. Because of the little amount of tissue in lung, there is also a low number of receptors, enzymes, and targets in general. This means that the signal-to-noise ratio (SNR) may be too low for the sensitivity of the available molecular imaging technology.
Quantitative and functional imaging-derived biomarkers for lung fibrosis
While evaluating and diagnosing the lung condition of patients is mainly a qualitative issue, animal research is in essence a quantitative discipline, requiring numerical data as readouts from all the examinations performed. Lung micro-CT and MRI are currently the most frequently used non-invasive modalities that deliver visual and quantifiable information about the whole lung and in three dimensions – including regional differences – with high resolution and sensitivity, yielding data that align well with imaging assessments routinely performed in lung disease patients. Moreover, quantitative measures derived from a single lung scan can provide insight into lung anatomy, function and pathology.
Pulmonary biomarkers such as the signal intensity and/or volume of the lungs, air spaces or lesions derived from micro-CT and MR images have been validated against gold standard histological measurements in several lung fibrosis models. For instance, quantification of changes in the volume of air in the lung from micro-CT data was validated to quantify progressive bleomycin- and adenoviral TGF-β1-induced lung fibrosis . A segmentation-based and fully automated algorithm was proposed, thus resulting in fast, easy and user-independent quantification . The aerated lung volume can alternatively be obtained via an algorithm based on the region growing, upon manual placement of a seeding point . The amount of air within the lungs is the biomarker that probably best corresponds to the vital lung function of the animal, similar to the parameters that can be measured by pulmonary function tests, such as tidal volume and total lung capacity. The interpretation of the functional lung biomarker that can be derived from the imaging data will depend on how the data were acquired, and during which phase of the breathing cycle. This depends in turn on whether acquisition was done four-dimensionally with retrospective gating on free-breathing animals (note that anaesthesia levels may have an influence here), or whether the animal was ventilated with acquisition done at maximum inspiration or at the end of expiration. Inflammation and fibrosis not only affect the air content of the lungs (represented by the hypointense voxels), but can also increase lung ‘tissue’ volume that includes any kind of lesion or airspace infiltration, for instance, collagen deposition in fibrotic disease, tumour growth or lung metastasis in cancer, inflammation and infection. A semi-automated delineation approach was proposed for quantification of tumour mass from micro-CT and MR images of a lung cancer mouse model . However, patches of inflammation and fibrotic lesions are not defined by clear boundaries, complicating contouring of the hyperintense voxels and hence calculation of their volume. To circumvent this, the entire lung can be manually or (semi-)automatically delineated to quantify the volume of hyperintense signals via overall thresholding within the total lung ( Fig. 3 ). Another approach is to calculate the mean of the signal intensities of all voxels inside the entire lung ( Fig. 3 ). This mean signal intensity represents the bulk changes in the air and tissue (including lesions) content of the lung. This approach was validated to provide an accurate readout for the extent of diffuse pathology such as lung fibrosis as detected by different MRI protocols and micro-CT . Defining the volume of the whole lung also generates a value for the total lung volume ( Fig. 3 ).
The importance of evaluating changes in this lung biomarker through fibrosis and lung disease progression in general has recently been highlighted. A large and significant increase in the total lung volume was revealed in the bleomycin-induced mouse model of lung fibrosis . It was demonstrated that this increase in the total lung volume must be taken into account for the interpretation of other lung biomarkers in this model, because quantifying disease only by the effect it has on the size of air spaces would underestimate the deposition of extracellular matrix during disease progression because of this marked expansion of the lungs as a whole . Quantification of different imaging-derived biomarkers for the lungs in their entirety is highly relevant for the translation of therapy evaluation results from mouse studies to human patients. Similar as that the increase in lung size that seems to compensate at least partially for the loss of airspaces due to fibrosis has been mainly overlooked, it may very well be that differences and imperfections in mouse models mimicking human disease account for at least some of the many discrepancies found when translating therapy evaluation results from mouse to man . Non-invasive imaging of both disease progression as the host response to it will play a crucial role in this regard.
Structural imaging of arthritis in small rodent models of arthritis
RA and OA are very different arthritic diseases in terms of pathophysiology, morbidity and treatment and the authors point the readers to specialised reviews for either pathology . However, from a structural imaging viewpoint, the choice of the imaging modality is dictated mainly by the characteristics of the tissue to be visualised (within the articular joint) rather than by the type of arthritis. The cartilage undergoes thickness decreases, focal lesions and/or superficial fibrillation both in RA and OA patients. The periarticular bone may become either severely resorbed in RA or hypermineralised (sclerotic) and with increased volume in OA. Bony outgrowths, called osteophytes, at the joint margins are also often present in both OA and RA. Severe inflammation of synovial membrane (synovitis) and its thickened appearance (synovial hyperplasia) are key signs of early RA whilst only occasionally observed in OA, albeit developing some abnormal calcification around the joint capsule. Although the focus of OA research has been originally on the articular cartilage, novel findings now indicate OA as a disease of the whole joint, with failure of different joint components . Many of these morphological changes observed in the articular joint of patients have been reproduced in the small rodent models of the OA and RA. While the pathogenesis of RA is understood to be initiated by a dysfunctional immune response causing severe inflammation of the synovium, the pathophysiology of OA is less clear, particularly on the roles of the different tissues in the joint in relation to their temporo-spatial contribution to the structural/compositional changes occurring in cartilage or bone . Improvements in clinical MRI have been able to demonstrate concomitant signs of pathology in subchondral bone and cartilage in patients with early OA, but this does not suggest the pathogenic one of the two tissues. Chemical or surgical animal models of OA might help answering this fundamental question with the help of an imaging technology matching the clinical MRI.
Magnetic resonance imaging of structural changes in articular joints affected by RA and OA
In vivo MRI of small animals is usually performed using dedicated equipment with higher magnetic field strengths ranging from 4.7T to 11T, which provide a higher resolution compared to clinical MRI scanners which rely on magnets that range from 1T to 3T. However, the acquisition of high-resolution MRI within an acceptable time frame remains an important drawback. In order to overcome the limited detection sensitivity and to increase the SNR, enhanced pulse sequences have been developed. These allow adequate anatomical areas to be imaged with scan times maintained within the limits of in vivo tolerance. As in clinical MRI, the MR sequence that best-delineated the cartilage from the surrounding tissues, that is, fat-suppressed (FS) T1-weighted three-dimensional (3D) gradient-echo (GE) sequence, has also been effective in determining quantitative morphological changes in the knee articular cartilage of a rat model . GE sequences with short echo time (TE) and relatively large flip angles provide T1-weighted images where the intra-articular fluid is less intense than the cartilage and fat is suppressed, therefore maximizing the contrast between cartilage, fluid and marrow, with cartilage showing a bright signal . 3D GE sequences have been successfully applied for the quantitative assessment of thickness and volume of cartilage in the larger rodent models of OA, including dog , guinea pig , rabbit and rat . However, in vivo MR imaging has been rarely used for mouse models of OA. This is because even the most advanced high-field MRI scanners cannot achieve sufficient spatial resolution to measure structural changes in the cartilage and bone of the mouse, whilst containing the scan time within limits compatible with longitudinal assessment . However, clinical and high-field MRI have been extensively utilised to monitor the synovial inflammation in small rodent models of inflammatory arthritis, including mouse models , due to the high sensitivity to fluid content in tissue. Generally, for imaging synovitis, MR T1-weighted images before and after intravenous gadolinium contrast are acquired using similar imaging protocols between experimental animals and patients. Another application of MRI is in the assessment of the loss of the macromolecular content of articular cartilage, which has been shown to occur before detectable macroscopic lesions. One of the main motivations behind this work has been the ongoing development of matrix drugs designed to slow or reverse the development of OA at this early stage. The two most commonly used techniques in experimental arthritis are delayed gadolinium-enhanced MRI of cartilage (dGEMRIC) and T2 maps. dGEMRIC works by allowing negatively charged gadolinium-diethylenetriamine pentaacetic acid (DTPA)-2 to distribute in cartilage in inverse proportion to the negatively charged glycosaminoglycans (GAGs) by diffusion. GAGs are a key cartilage matrix component required for cartilage hydration and swelling, and T1 maps of the distribution of Gd-DTPA-2 reflect the underlying glycosaminoglycan content. GAG loss in the knee cartilage of a rabbit mode of immobilisation-induced OA was monitored weekly for 3 weeks and found to strongly correlate with histological findings . T2 maps of articular cartilage are a function of the water content of the tissue. Measurement of the spatial distribution of the T2 may reveal areas of increased or decreased water content, which correlate with cartilage damage. Focal increases in T2 within cartilage have been associated with matrix damage, particularly loss of the collagen matrix. Recent application of T2 mapping in longitudinal studies, either in rat and rabbit models of OA or in a rabbit model of allograft chondrocyte implantation repair demonstrated high sensitivity to early degenerative and reparative changes in cartilage matrix, respectively.
In two recent studies, high field MRI and micro-CT imaging were combined to assess longitudinally the progression of bone marrow lesions (BMLs) in a surgical rat model of OA . The authors showed that in the early phases of disease, MRI images showed BMLs which were not visible in micro-CT images. However, micro-CT was required to determine the bone mineral density of bone in the areas detected by the MRI in order to determine the nature of such BMLs in relation to bone tissue compositional changes. This example supports the importance of integrating complementary imaging modalities in the preclinical setting in order to draw a comprehensive picture of the joint pathology which is not merely limited to the assessment of the articular cartilage and synovium.
Micro-CT of structural changes in articular joints affected by RA and OA
Micro-CT is, at present, the gold standard imaging technology to quantify morphological changes in the mouse skeleton. Current commercial desktop specimen scanners (for ex vivo imaging) have the capability to visualise 3D bony structures at submicron resolutions, including trabecular bone , subchondral bone , focal erosions and compact bone vascular and lacunar porosity. However, the current in vivo micro-CT scanners for non-invasive imaging studies cannot resolve details <5–10 microns (approximately one order of magnitude less than that of ex vivo scanners). Such reduction in performance is due to different system design requirements, which must compromise on SNR in order to limit radiation exposure. Thus, structural measurements performed by in vivo micro-CT at a lower resolution have been often compared to and validated by ex vivo micro-CT measurements at higher resolution referred to as the gold standard. Notwithstanding these limitations, in vivo micro-CT imaging has been successfully employed to monitor bone structural and compositional changes in rat and mouse models of OA and rat mouse models of RA.
Several 2D and 3D structural parameters in bone tissue, such as volume, thickness, trabecular connectivity and bone mineral density can be measured by semi-automated procedures which require the manual contouring of a volume of interest to be segmented and evaluated by standard software programmes. Interestingly, it has been demonstrated that the porosity of subchondral bone – determined non-invasively – became increasingly elevated in proximity of the zones of cartilage with more severe lesions, which suggests a direct interaction between the two tissues .
However, the evaluation of some pathological features of arthritis such as focal erosions in small rodents has been performed predominantly by conventional qualitative histomorphometry with few attempts to determine a quantitative measure. In the CIA mouse model of RA, paw and knee periarticular bone display an increasing pitted surface as severity increases, which is demonstrated very clearly by 3D rendering of the joint (showing the external surfaces of periarticular bone, Fig. 4 ). Surface roughness, increase by focal erosions, was evaluated by computing the ratio of the bone volume (BV) over the area of bone surfaces (BS) including such volume, with the assumption that, for a relatively constant volume, focal erosions would increase the surface area and therefore their ratio (BS/BV). More recently, a different parameter was proposed which is not biased by the assumption of a constant BV. Based solely on the assumption that non-arthritic animals have smoother periarticular bone surfaces compared to arthritic, the new parameter was calculated as the ratio of the computationally smoothed bone surfaces BS smooth over the original BS with values of BS smooth /BS close to one for perfectly smooth surfaces and close to zero for extremely rough surfaces. Interestingly, this new parameter BS smooth /BS allowed to demonstrate that the administered drug had no effect on superficial focal erosion of periarticular bone, in opposition to the old parameters BS/BV which indicated a beneficial effect due to the bone anabolic effect of the treatment which had expanded its volume ( Fig. 4 ). This example demonstrates the importance of developing (or continuously updating) unbiased quantitative parameters to improve the sensitivity to structural changes and the specificity of image-based endpoints. Ultimately, improved unbiased quantitative parameters will help reducing the number of experiments required to draw conclusions about pharmacological targets.
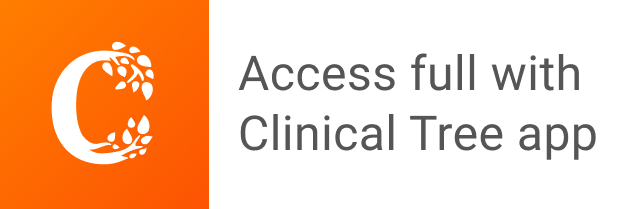