Abstract
There is an overwhelming need for a simple, reliable tool that aids clinicians in diagnosing, assessing disease activity and treating rheumatic conditions. Identification of biomarkers in partially understood inflammatory disorders has long been sought after as the Holy Grail of Rheumatology. Given the complex nature of inflammatory conditions, it has been difficult to earmark the potential biomarkers. Metabolomics, however, is promising in providing new insights into inflammatory conditions and also identifying such biomarkers. Metabolomic studies have generally revealed increased energy requirements for by-products of a hypoxic environment, leading to a characteristic metabolic fingerprint. Here, we discuss the significance of such studies and their potential as a biomarker.
Introduction
The study of metabolism in disease has been an area of interest for many centuries. For example, ancient Chinese doctors in 1500 BC would use ants to detect glucose in urine to aid the diagnosis of diabetes mellitus . Interventions with metabolic consequences, such as phlebotomy, were widely used from the time of Galen until the 19th century. These may have been rationalised as a result of works such as the Nature of Man in the Hippocratic corpus, which suggested that the proper course to take during epidemics was to keep the body as thin and weak as possible .
The musculoskeletal system is very active metabolically, with skeletal muscle, turnover of bone and other structural components having high requirements for energy and substrates. Inflammation adds to these demands as in rheumatoid arthritis (RA) the resting energy consumption is increased by approximately 8% compared to controls. Interestingly, a similar further increase is observed in patients who smoke. As smoking is a known risk factor for the development of seropositive RA, this suggests that the metabolic changes associated with disease may be of significance . Although the mechanism for the increased metabolic rate in RA is yet to be elucidated, it is reasonable to postulate that the inflammatory process is a major contributor. The fact that joints are hot in inflammatory arthritis is a strong indicator of increased local metabolism. This has been visualised using positron emission tomography (PET) scans, which rely on the enhanced uptake of a labelled but nondegradable derivative of glucose (18-F-deoxyglucose) that accumulates in joints in RA patients , thus indicating enhanced glycolytic activity. Inflammation also drives other processes such as the decrease in muscle mass and increased fat tissue, in a process known as rheumatoid cachexia, which involves significant alterations in systemic metabolism. PET imaging has also been reported to identify such enhanced metabolic activity in extra-articular sites, in particular subcutaneous nodules and lymph nodes , thereby indicating increased immune metabolism. The liver is also a source of increased metabolism as the inflammatory response leads to the hepatic production of acute phase proteins and the likely enhancement of the Cori cycle to clear excess blood lactate produced in inflammatory sites, which is certainly observed in the more acute inflammation seen in burns . Changes in metabolism may be seen before the development of clinical disease, for example, decreases in blood lipids are an early feature , which may be modified following treatment . These local and systemic metabolic consequences of RA suggest that the investigation of metabolism may prove useful in diagnosis and in assessing responses to therapy.
Principles of metabolomics
While changes in individual metabolites have been reported in inflammatory conditions, in recent years, metabolomics has been used to provide a generalised outline of broader metabolite profiles. Metabolomics is the new kid on the “omics” block. While genomics studies the genetic basis of phenotype, and transcriptomics and proteomics study the products of these genes, metabolomics seeks to identify the downstream effects caused by the action of these enzymes and proteins in the context of metabolic activity and management. Utilising a hypothesis-forming methodology, it is propelled by the non-discriminant analysis of the low molecular weight metabolite constituent of target samples.
Small molecules (<1500 Da) are quantified within compartments (synovial fluid, blood, urine, saliva, tears, cerebrospinal fluids and intact cells) and metabolite profiles or fingerprints are generated, which may contain thousands of metabolites. The metabolites are recognised and measured using mass spectrometry or nuclear magnetic resonance (NMR) spectroscopy. The derived data result in spectra containing a number of peaks, signifying proton resonance in proton NMR or mass/charge ( m / z ) ratios in mass spectrometry . Metabolites are recognised by referencing to the metabolite databases or by direct metabolite assay. The Human Metabolome Database lists >41,000 metabolite entries in the latest version; however, only 3000 have been associated with diseases to date . In common with genomic, proteomic and transcriptomic approaches, metabolomic experiments produce large bodies of data often from multiple samples, necessitating the use of multivariate analysis to simplify and extract meaning from the resulting data.
Principles of metabolomics
While changes in individual metabolites have been reported in inflammatory conditions, in recent years, metabolomics has been used to provide a generalised outline of broader metabolite profiles. Metabolomics is the new kid on the “omics” block. While genomics studies the genetic basis of phenotype, and transcriptomics and proteomics study the products of these genes, metabolomics seeks to identify the downstream effects caused by the action of these enzymes and proteins in the context of metabolic activity and management. Utilising a hypothesis-forming methodology, it is propelled by the non-discriminant analysis of the low molecular weight metabolite constituent of target samples.
Small molecules (<1500 Da) are quantified within compartments (synovial fluid, blood, urine, saliva, tears, cerebrospinal fluids and intact cells) and metabolite profiles or fingerprints are generated, which may contain thousands of metabolites. The metabolites are recognised and measured using mass spectrometry or nuclear magnetic resonance (NMR) spectroscopy. The derived data result in spectra containing a number of peaks, signifying proton resonance in proton NMR or mass/charge ( m / z ) ratios in mass spectrometry . Metabolites are recognised by referencing to the metabolite databases or by direct metabolite assay. The Human Metabolome Database lists >41,000 metabolite entries in the latest version; however, only 3000 have been associated with diseases to date . In common with genomic, proteomic and transcriptomic approaches, metabolomic experiments produce large bodies of data often from multiple samples, necessitating the use of multivariate analysis to simplify and extract meaning from the resulting data.
Making sense of the data
Statistical analysis of the metabolomic data can be divided into directed and undirected approaches . Undirected analysis includes principal component analysis (PCA), wherein the aim is to describe the maximum variation in the data set without supplying information to the model about the class groupings. The end result is a series of orthogonal principal components (i.e., sets of variables that vary together but independent of the other sets), describing the range of variations in the data through weighted peaks. If the source of greatest variation in the data is the difference between control and test samples, PCA alone is enough to distinguish class groupings . Clinical research tends to deal with more variable samples, and thus confounders may conceal the true variances in the samples. Directed or supervised analysis techniques, however, may prove to be more suitable in such situations. Partial least squares regression (PLSR) may be used to explain variation in one data set by referring to another, for example, using metabolite concentrations to predict outcome, progression or severity. Furthermore, PLS discriminant analysis (PLS-DA) uses multivariate metabolomic peak data to describe the assignment of samples to binary cohorts. In essence, the technique focuses on pinpointing the variation that describes the differences between control and test samples . This makes it feasible to extract the clinically pertinent variation in the face of substantial unrelated noise. The outputs of these methods aim to produce statistical models from which it is possible to precisely forecast disease state or patient group from biological samples. However, the models in question are likely to be complex and include multiple correlated peaks. Forward selection regression analysis may therefore be utilised to iteratively remove peaks resulting in a small number of metabolites while preserving predictive proficiency, making it ideal for cheaper, more specific bioassays. Selection of controls is vital, and controlling modifiable factors such as smoking, medications and diet is crucial to the formulation of accurate models .
Inflammation and metabolism
The strong relationship between metabolism and inflammation can be exemplified by cachexia, the loss of cellular mass associated with disease. Tumour necrosis factor (TNF) alpha, also known as ‘cachexin’, is a central cytokine in cachexia. RA is associated with systemic chronic inflammation and with rheumatoid cachexia. Muscle wasting in rheumatoid cachexia is a shared feature with classical cachexia, but low body mass index (BMI) is unusual as the fat mass is maintained or increased . Cytokines, such as TNF, interleukin (IL)-1 and IL-6, are hypothesised to be responsible for the muscle loss observed in rheumatoid cachexia. The ubiquitin–proteasome pathway is activated by TNF, which is responsible for proteolysis of tissues. Pro-inflammatory cytokines may inhibit protein synthesis in response to nutritional intake, the so-called anabolic resistance; hence, rheumatoid cachexia-induced muscle wasting is related to RA disease activity .
In self-limiting inflammatory disease, the presence of local factors may prevent the recruitment or release of additional leukocytes, which abates the inflammation . By contrast, an excess of pro-inflammatory mediators is thought to drive acute inflammation into a chronic inflammatory state.
The aetiology of inflammation
Inflammatory diseases are a heterogeneous group of conditions usually involving both localised and systemic inflammation. Although many factors have been implicated in perpetuating chronic inflammation, the exact mechanism of how acute inflammation progresses to a chronic state is unknown. However, an interplay between genetic and environmental factors is becoming increasingly apparent, which plays an important role .
Genome-wide association studies (GWASs) have identified genetic polymorphisms that increase the risk of acquiring particular conditions. Human leukocyte alleles (HLAs) are vital in antigen recognition; in addition to the peptidylarginine deiminase type IV (PAD14) gene, which controls the production of cyclic citrullinated proteins (CCPs), these have been implicated in the development of RA . However, like RA, the aetiology of many inflammatory conditions is not fully explained by genetics alone, and thus the role of the environment should be considered.
Smoking plays an important environmental role in the development of a number of inflammatory conditions. Smoking activates an acute inflammatory response and generates a considerable amount of reactive oxygen species (ROS) . Lipopolysaccharide in cigarette smoke may be responsible for initiating an undesirable immune response resulting in chronic inflammatory disease . Reduced circulating antioxidants in smokers suggest an increase in ROS, which in turn creates a pro-oxidant environment, thus multiplying the probability of oxidative damage to the integral cellular components . Smoking appears to play a fundamental role in developing RA in individuals with susceptibility genes such as HLA-DRB1 (human leukocyte antigen-D related B1) and PTPN22 (protein tyrosine phosphatase non-receptor type 22) , and oxidative processes may also play a role. The serum and lymphocyte levels of major antioxidant reduced glutathione (GSH) decreased by around 40% in RA patients compared to controls . This metabolic change has consequences for leukocyte function, as the protein tyrosine phosphatase CD45, critical in partnership with PTPN22 in regulating lymphocyte signalling, is oxidatively inactive in these RA cells , which has an impact on their subsequent function and responses , and may contribute to the well-documented aberrant responses of these cells in RA . Interestingly, a similar decrease in GSH levels is observed in the healthy elderly with parallel effects on CD45 oxidation and function . Given that RA manifests itself largely in older adults, such age-related changes in antioxidant metabolites and immune function may contribute, together with smoking, as an important non-genetic, environmental driver of disease.
Inflammatory mediators
Cytokines TNF-α and IL-1 promote inflammatory responses by inducing cartilage degradation and a cell-mediated immune reaction. Other key players implicated in destructive inflammation include cyclooxygenase (COX)-2, migration inhibition factor, interferon gamma and matrix metalloproteinases . On the contrary, cytokines such as IL-4, IL-10 and IL-13 act to induce anti-inflammatory effects .
A number of cytokines participate in inducing inflammatory responses that are not disease specific. For instance, C-reactive protein (CRP) is secreted by a number of different cell types and it directly stimulates immune cells. CRP has therefore been associated with local and systemic inflammatory response . In addition, adipokines (or adipocytokines), as the name suggests, are inflammatory cytokines secreted by white adipose tissue that can induce inflammation by binding to discriminatory transmembrane receptors .
Metabolic inflammation
A number of factors influence inflammatory response, namely immunological, microbiological and toxic agents, which act by stimulating humoral and cellular intermediaries. Lipid mediators and interleukins are secreted in excess in early inflammation, thus playing a vital role in inducing organ dysfunction. Inflammatory activity releases arachidonic acid, which is metabolised to prostaglandins and leukotrienes. The interest in lipid mediators as therapeutic targets has led to multiple attempts at regulating their production. This includes blockade of COX and lipoxygenase pathways, phospholipase A2 inhibition (enzyme responsible for AA (arachidonic acid) release), blockade of platelet activating factor and leukotriene. These attempts have not been uniformly effective, but they have shown some promise in certain inflammatory disorders such as multiple-organ dysfunction in sepsis, RA and asthma. Dietary supplementation with long-chain fatty acids such as eicosapentaenoic acid (EPA) holds some promise theory. Endogenously secreted EPA competes with AA for enzymatic metabolism, thus generating less inflammatory and chemotactic products .
Environment plays an important role in the pathology of inflammation. Hypoxia appears to be a common environmental theme conducive for fostering inflammation across a number disease processes such as trauma, malignant tumours, bacterial infections and autoimmunity . Low oxygen in tissues may have profound effects on tissue and immune cell metabolism and function and may be an important factor in driving persistence .
Use of metabolomics in inflammatory diseases
Systemic inflammation has a large impact on metabolism; thus, subsequently, metabolomics have been utilised to study human and animal models of inflammation. These studies have shown that metabolite levels are modified by inflammation, which has offered valuable insights into the pathophysiology of these conditions and exposed numerous possible biomarkers for disease assessment. NMR and mass spectroscopy have been utilised in building disease metabolite profiles, which have been useful in a number of instances including distinguishing between patient groups or identifying responses to therapy .
In the following sections, we examine the number of key markers that have been identified by metabolomic studies to date, followed by disease-specific metabolomic findings.
Lactate and hypoxia
As previously mentioned, hypoxia and lactate are important factors when considering inflammation. In the absence of hypoxia cells, glucose metabolise through the tricarboxylic acid (TCA) cycle to produce nicotinamide adenine dinucleotide (NADH), which provides the substrate for oxidative phosphorylation resulting in 36 adenosine triphosphate (ATP) molecules per glucose molecule . In the presence of hypoxia, pyruvate is excreted as lactate, with a reduction of two ATP molecules per glucose in the cell. Physiological oxygen concentrations vary amongst tissues ranging from 5% to 12%. Lymph nodes, the eye and synovium are tissues that operate at the lower end of oxygen concentration due to either reduced vascularisation or perfusion .
Local hypoxia can occur due to various reasons. Blood vessel constriction or demands from highly active inflamed tissues with an increased cellularity exceed supply. Furthermore, circulating phagocytes can impede blood flow into the site of inflammation .
In the context of malignancy, the environment is known to be hypoxic with extensive angiogenesis, necessitating an increased oxygen supply to the tissue. The synovial fluid of patients with RA has shown lower partial pressures of oxygen compared to that in osteoarthritic and traumatic injuries . In fact, hypoxia appears to exhibit a directly proportionate relationship with synovitis in RA . Fibrotic dermal tissue in systemic sclerosis patients is also found to have lower oxygen concentration than normal tissue in the same patients and controls . Oxaloacetate and urea levels are elevated in vitreous fluid of patients with uveitis, which is readily explained as by-products of macrophage anaerobic respiration . Cellular oxygen recognition mechanisms are used by cells to make necessary adjustments. Hypoxia-inducible factor (HIF), a transcription factor, is responsive to oxygen levels. HIF is actively reduced in oxygen-rich environments. HIF expression is suggestive of hypoxic conditions and has been detected in RA and multiple sclerosis . A subtype of HIF, HIF-1a, has been shown to be important for macrophage aggregation, invasive, motility and ability to kill bacteria .
HIF also has a significant effect on cellular metabolism. HIF induces glycolytic enzymes, which drives glycolytic metabolism preferentially over oxidative phosphorylation . Thus, ATP production continues despite inadequate oxygen, albeit at the expense of efficiency per molecule of glucose .
Hypoxia and HIF stabilisation greatly influence immune cells. Peripheral blood CD4 + T cells placed under hypoxia lead to the induction of genes involved in metabolism and homeostasis . Innate immune cells provide possible evolutionary evidence that inflammation is best served in a hypoxic environment. Macrophages and neutrophils favourably utilise glycolysis to supply ATP even at higher oxygen levels . Hypoxia appears to activate tissue-resident macrophages, and hypoxic sites of chronic inflammation collect macrophages . Macrophages in the hypoxic surroundings are accompanied by upregulation of an array of proinflammatory cytokines such as IL-1 , IL-6 , interferon (IFN) gamma and TNF-α .
Lactate is predominately a product of anaerobic respiration, namely as a result of the action of lactate dehydrogenase on glycolytic pyruvate. Surplus cellular lactate is excreted, converted in the liver by the Cori cycle to glucose and recirculated. Alternatively, if adequate oxygen is present, intracellular NADH may be used to convert lactate into pyruvate, which can then be recycled in the citric acid cycle. However, insufficient oxygen supply leads to the accumulation of lactate. HIF-induced upregulation of lactate dehydrogenase A compounds this accumulation .
Lactate therefore is a significant indicator of inflammatory sites under hypoxia. However, this relationship is not only observed in hypoxic conditions. Cancer cells preferentially metabolise glucose by glycolysis independent of the oxygen supply, the so-called Warburg effect . It has been hypothesised that replicating and differentiating cells must use the same mechanism in order to manufacture the necessary biomass for cellular replication. Thus, glucose and glutamine can provide the bulk of carbon, nitrogen and energy for cell growth and division. Under growth conditions, it is inefficient for glucose to be metabolised by oxidative phosphorylation . Thus, non-proliferative ATP-dependent organs such as the brain prefer oxidative phosphorylation of glucose. In comparison, under resting conditions, skeletal muscle largely metabolises fatty acids, switching to glycolysis and oxidative phosphorylation during activity . In proliferative tissues such as lymph nodes, there is a predisposition toward metabolising glucose to lactate, pyruvate and carbon dioxide, and glutamine to ammonia, glutamate and aspartate. This may relate to tissues and cells, such as polymorphonuclear leucocytes, that invade inflammatory sites, compounding lactate production .
Lactate as a marker of inflammation has been noted clinically across an array of conditions: from elevated lactate levels in the synovial fluid of RA patients to elevated lactate levels in the cerebrospinal fluid of multiple sclerosis patients and idiopathic intracranial hypertension patients . The downstream effects are also observed in inflammatory states as excess pyruvate is converted to intermediates of the citric acid cycle, malate and oxaloacetate . Elevated urine malate levels are a strong predictor of disease activity in the Hartley guinea pig model of osteoarthritis (OA), which is increasingly being seen as a condition with significant systemic inflammatory response .
Alternative energy sources
Organ-specific metabolic rates and favoured substrates are well known, with the heart and kidney consuming the chief share of energy in a resting adult . These basal rates are susceptible to substantial variation by a number of factors including malnutrition, smoking, illness and inflammation . A study showed that the basal rate of RA patients was 8% higher than the healthy control group. This increased to 20% in the RA patients who smoked . Inflammation is a vastly energy-dependent process with an acute need for sufficient energy supply . Fever is accompanied by an 11% upsurge in energy consumption per 1 °C, whereas phagocyte production during an infection consumes almost 790 kJ . In sepsis, the metabolic rate can increase in the range of 30–60% above baseline . The contrary also applies, with energy surplus states related to metabolic syndrome and obesity propelling modified immune response and chronic inflammation .
Thus, accessible energy plays a vital role in immune activation and resolution. Resting requirements for non-brain and non-proliferative tissues are principally met by the oxidation of free fatty acids . The use of fatty acids is normally earmarked in well-perfused tissues due to their oxidation requirement. However, damage to the joint in inflammatory and OA seems to be associated with infiltration of fatty acids into the synovium. In OA, increased lipoprotein-associated fatty acids, together with metabolism products such as glycerol, ketones and pyruvate, are all suggestive of lipolysis being an energy source. Similarly, reduced chylomicron and triglycerides associated with very low-density lipoproteins in RA synovial fluid compared to controls suggest an enhanced use of fats, an energy source in the joint despite the hypoxic environment. Blood plasma levels of lipids and acetylated glycoprotein were also elevated in RA .
The kidney and liver release ketone bodies into the circulation after breaking down fatty acids. Ketone bodies such as acetoacetate and 3-hydroxybutyrate deliver an essential energy source for the heart and brain, by reconversion to acetyl-coenzyme A (acetyl-CoA) and re-entering the citric acid cycle in the tissues, while acetone is expelled as waste. In fasting conditions, plasma levels of ketone bodies are low, as tissues with lower metabolic needs sustain themselves on local reserves of fatty acids; however, these sources are insufficient for dynamic, high energy-dependent tissues and ketone body production increases significantly. Changes in ketone body metabolism have been detected in inflammatory diseases in the absence of glucose restriction. Chronic inflammation may cause metabolic changes, especially in the context of cachexia-associated catabolism and ketogenesis. The presence of ketone body 3-hydroxybutyrate in urine has been linked to respiratory chain deficiency leading to impaired NADH oxidation .
Tissue degradation and waste
In inflammatory states, the normal tissue turnover of cellular and acellular biomass is disrupted, which offers an opportunity to identify relevant biomarkers. Specific tissue-derived metabolites may provide the most transparent evidence of tissue destruction. For instance, hyaluronic acid is a major component of articular cartilage proteoglycan aggregates and is critical for the structure of extracellular matrix. It appears to correlate well with joint destruction in RA patients .
Essential amino acids also show promise in this regard. As they cannot be synthesised de novo, assuming a stable diet, variations in concentration must be attributable to catabolism of current proteins derived from tissue destruction, apoptosis or cellular autophagy. In a study comparing faecal extracts from patients with ulcerative colitis (UC), Crohn’s disease (CD) and controls, the elevated levels of alanine, isoleucine, leucine, lysine and valine were all observed in patients with CD. This is most likely as the disease affects the whole intestine . Mouse models of CD showed alterations in urinary metabolites relating to tryptophan metabolism . This seems to be a universal characteristic of inflammatory processes, as demonstrated using adenoviral vector to stimulate chronic expression of inflammatory IL-1B or TNF-α; the IL-1B-treated group similarly observed increases in leucine, isoleucine, valine, n-butyrate and glucose levels . Interestingly, dietary supplementation with branched chain amino acids (BCAAs), a subset of essential amino acids, has been shown to drive an increase in Th1-like responses via IL-1, IL-2, TNF and interferon, signifying the likelihood of an association between local release from degradation and chronic inflammation .
Catabolism of protein leads to a build-up of ammonia. This is converted to urea predominately in the liver and kidney, for urinary excretion . Excretion of nitrogenous waste products is the key to homeostasis; nevertheless, overproduction or poor circulation can allow accumulation of these metabolites, For example, patients with lens-induced uveitis generate considerably increased urea, glucose and oxaloacetate in vitreous humour compared to patients with chronic non-infectious uveitis . Furthermore, a study of urinary metabolites in a mouse model of inflammatory bowel disease revealed an increase in trimethylamine compared to controls running parallel to the progression of IBD (inflammatory bowel disease) , a finding substantiated in human studies using both faecal and urinary samples .
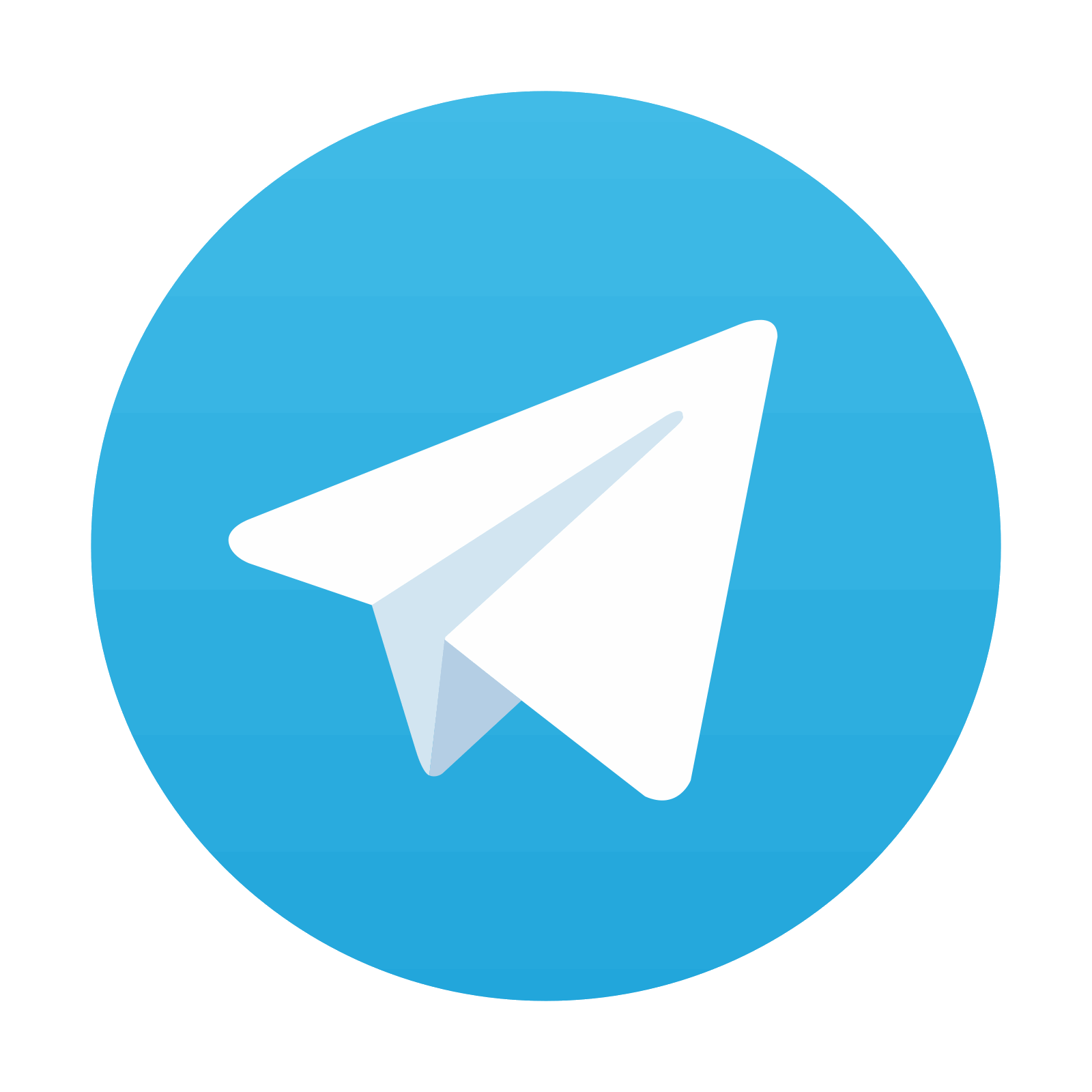
Stay updated, free articles. Join our Telegram channel
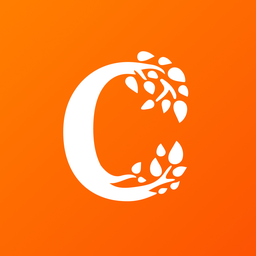
Full access? Get Clinical Tree
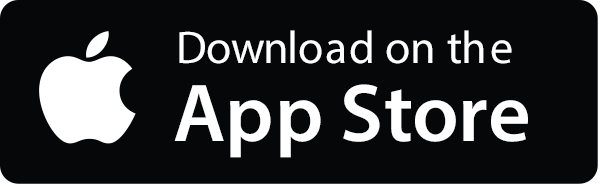
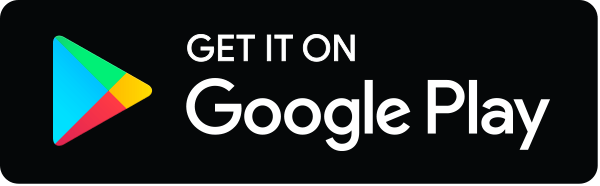