INTRODUCTION
Chronic pulmonary disease has a negative effect on several patient-centered outcomes. Among these, exercise capacity, dyspnea, and quality of life are the most relevant. Analysis of factors that contribute to exercise limitation, exercise-induced dyspnea, reduced quality of life, and pathophysiologic changes in patients with chronic pulmonary disease underlies research efforts to identify effective interventions for this patient population.
As defined by the American Thoracic Society and the European Respiratory Society, pulmonary rehabilitation is an evidence-based, multidisciplinary, and comprehensive intervention for patients with chronic respiratory diseases who are symptomatic and often have a decreased ability to participate in daily life activities. Integrated into the individualized treatment of such patients, pulmonary rehabilitation is designed to reduce symptoms, optimize functional status, increase participation, and reduce health care costs by stabilizing or reversing systemic manifestations of the disease. Comprehensive pulmonary rehabilitation programs include patient assessment, exercise training, education, nutritional support, and psychosocial support.
This treatment approach has clearly demonstrated favorable and long-lasting effects on all patient-centered outcomes. In addition, pulmonary rehabilitation appears to have positive effects on other important outcomes (eg, number and severity of exacerbations, health care resource utilization, and survival) in patients with chronic pulmonary conditions, particularly those with chronic obstructive pulmonary disease (COPD). Although the evidence for the efficacy of pulmonary rehabilitation is strong and it is highly recommended by current guidelines, only a minority of eligible patients with chronic pulmonary disease are included in rehabilitation programs. This discrepancy may derive from lack of belief in the efficacy of such programs, lack of local access, or concerns about cost. The first of these impediments can be addressed by intensified promotion of the beneficial effects of pulmonary rehabilitation in the medical community; the other two, however, may require the design of simple and locally available programs using a minimum amount of resources that still produce clinically relevant effects.
OVERVIEW OF THE NORMAL LUNG
The primary function of the lung is ventilation–perfusion matching to maximize delivery of oxygen to and removal of carbon dioxide from the tissues. Air enters the respiratory system through the trachea, which then subdivides into the cartilaginous primary bronchi. These bronchi divide into secondary bronchi, supplying each lobe of the lung; the secondary bronchi branch into segmental bronchi (or tertiary bronchi); and these tertiary bronchi subdivide into terminal bronchioles.
At the level of the bronchioles, the lining has lost its cartilage and is now predominantly epithelial tissue with smooth muscle. However, gas exchange does not occur until the terminal bronchiole subdivides again into respiratory bronchioles, containing the alveolar ducts and sacs. The predominant cell type in the alveoli is the type I alveolar cell, which allows for diffusion of oxygen and carbon dioxide. These cells comprise about 90% of the alveolar cells in the lung. The other 10%, type II cells, produce surfactant, which is needed to reduce the surface tension of the lung and allow for maximal gas exchange.
During normal inspiration, the diaphragm contracts and pulls down the contents of the abdominal cavity. At the same time, the external intercostal muscles pull the thoracic rib cage forward. These mechanisms combine to increase the volume of the thoracic cavity. According to Boyle’s law (which states that pressure is inversely related to volume), the increase in volume produced by these two maneuvers must be balanced by a decrease in pressure. It is this negative pressure that pulls air into the airways. In contrast, normal exhalation is by definition a passive process. Exhalation is determined by the elastic recoil of the lung and can be changed to forced exhalation with contraction of the internal intercostal muscles. The abdominal muscles (eg, external and internal obliques, rectus abdominis, and transversus abdominis) can also be used for forced exhalation. Accessory muscles that can be used for inhalation include the pectoralis, serratus anterior, and sternocleidomastoid.
Perfusion to the lungs is not uniform, given the pressure differences in the alveoli, arteries, and veins that exist from the apex to the base of the lung. A major reason for this difference is gravity, which leads to a higher-pressure blood supply at the base of the lung. Three perfusion zones, referred to as West’s zones, are differentiated (Figure 24–1). Zone 1, located at the apex of the lung, achieves the least amount of perfusion; the alveolar pressure (Pa) here exceeds the arterial (PA) and venous pressures (Pv), causing the arteries and veins to collapse. This leads to a significant amount of dead space at the apex of the lung. In zone 2, located in the middle third of the lung, the PA is greater than the Pa, but Pv is still less than Pa. This causes the venous vessels to collapse while the arteries remain open. Consequently, more perfusion occurs, but not as much as would occur were the venous system allowed to operate at full capacity without collapse. Zone 3, the point of maximal perfusion, is located at the base of the lung. In this zone, the PA and Pv exceed the Pa; therefore, the vessels do not collapse and maximal perfusion is achieved.
The ventilation capability of the lung is very similar to its perfusion capability. The alveoli at the apex of the lung are often overstretched and therefore receive less ventilation. However, perfusion is less than ventilation at the apex of the lung, despite both being reduced; therefore, the ratio of ventilation to perfusion (V/Q) is still greater than 1 (estimated at ∼3). Moving downward through the lung, ventilation increases but perfusion increases to a greater extent, resulting in a V/Q ratio less than 1. This functional difference has clinical relevance because often changes occurring in the base of the lung have a very large impact on overall gas exchange.
Lung compliance is defined as the change in volume of the lung in relation to the change in pressure. Lung compliance is dependent on elastic recoil; the less compliant the lung is, the smaller the volumes that can be attained with a given amount of pressure and the more dyspneic a patient can feel. Conversely, if lung compliance is too great, a large enough pressure for exhalation cannot be generated given the overstretching of the lung that can occur. Restrictive lung diseases are associated with reduced lung compliance and, therefore, reduced total lung capacities. Obstructive lung diseases, however, often are caused by destruction of the airway (eg, as in emphysema), resulting in increased compliance. Age also causes an increase in airway compliance.
EVALUATION OF LUNG FUNCTION
Pulmonary function testing is a well-established method of differentiating between obstructive and restrictive lung diseases, following the progression of such diseases, and differentiating lung disease from other possible causes of dyspnea and other respiratory complaints. The key measurements and calculated values that are extracted from pulmonary function testing (Figure 24–2) include total lung capacity (TLC), vital capacity (VC), residual volume (RV), tidal volume (Vt), functional residual capacity (FRC), and inspiratory and expiratory reserve volume (IRV and ERV, respectively). Other values that can be obtained include forced expiratory volume in 1 second (FEV1), forced vital capacity (FVC), and forced expiratory volume in 6 seconds (FEV6). These values are obtained through spirometry, which provides a cost-effective method of measuring and observing changes in specific components of lung function.
Figure 24–2
Pulmonary functions tests (PFTs) are noninvasive diagnostic tests that provide measurable feedback about the function of the lungs, evaluating and calculating values for total lung capacity (TLC), vital capacity (VC), residual volume (RV), tidal volume (Vt), functional residual capacity (FRC), and inspiratory and expiratory reserve volume (IRV and ERV, respectively).
Spirometry is performed using a calibrated instrument; patients are instructed to maximally inhale (from Vt to VC) and then maximally exhale (to RV) using all their force. This maneuver is repeated three times, and the highest values (assuming relative reproducibility) for FEV1 and FVC (or FEV6, depending on institutional preference) are documented. As defined, FEV1 is the amount of air, and theoretically the largest proportion of air, that is exhaled within the first second. This is a reflection of the mechanics of the large and medium-sized airways. Today FEV6 is increasingly being utilized, replacing FVC, as it makes it easier to identify the “end of expiration,” given a fixed value of 6 seconds, and additionally results in less participant fatigue and greater reproducibility. In patients with obstructive lung disease, the FEV1 is significantly reduced compared with the FVC or FEV6, and the ratio is reduced below the accepted threshold of 0.7 or 70% of predicted value. In patients with restrictive lung disease, however, both the FEV1 and FEV6 are reduced substantially in proportion and the ratio remains normal (75–80%) or even elevated.
Two methods are used to measure lung volumes: the gas dilution method and body plethysmograph. The gas dilution method involves using either an inert gas (eg, helium) or a method called nitrogen washout to calculate FRC. In the helium method, the patient is connected to a spirometer attached to a fixed concentration of helium in a fixed volume. The patient is allowed to breathe normally at normal Vt respirations until equilibrium is reached between the helium and the air in the patient’s lungs. Because the unit is a closed circuit, the law of conservation of matter dictates that the volume multiplied by the fraction of helium present beforehand must equal the volume multiplied by the concentration of helium afterward. The volume present afterward is the total volume of the lung’s FRC and the spirometer, and subtracting the volume of the spirometer therefore gives the FRC value. This value can then be used to calculate the TLC.
The other method, body plethysmography (Figure 24–3), involves placing the patient in an enclosed box with a fixed, known volume. The patient is instructed to pant against a closed shutter, which theoretically produces pressure that is proportional to the FRC of the lungs. This value can then be used to calculate the TLC and other lung volumes, as described previously. TLC is generally most useful in calibrating treatment for patients with restrictive lung disease. In patients with normal or even elevated FEV1/FVC ratios, determination of the TLC can help to confirm whether this is a normal variant or represents restrictive physiology. TLC that is normal or elevated essentially rules out restrictive lung disease, whereas reduced TLC points toward a restrictive pattern.
The diffusion lung capacity for carbon monoxide (DLCO) aids in further defining specific obstructive or restrictive patterns of disease. The test is performed by having the patient take in a single breath of 0.3% carbon monoxide and 10% helium, hold it for 10 seconds, and then exhale quickly for no more than 4 seconds. The subsequent concentration of carbon monoxide is measured and calculated. This is compared with standardized normal values; severe reduction in DLCO is defined as a value that is less than 40% of predicted.
The DLCO can help differentiate among likely causes in patients with obstructive lung disease. Significant reduction in DLCO is associated with emphysema. However, in chronic bronchitis-type COPD, patients often have a normal DLCO. Similarly, in patients with restrictive lung disease, the DLCO can help in differentiating among intrinsic and external causes. Intrinsic causes include lung diseases such as sarcoidosis and pulmonary fibrosis; external causes include restriction resulting from conditions such as obesity, kyphoscoliosis, and neuromuscular disease. If the patient’s DLCO is reduced, intrinsic lung disease is most likely causing the restrictive pattern. If normal, it points toward an external cause of the restrictive pattern, which should be investigated more thoroughly.
CLASSIFICATION OF LUNG DISEASE
ESSENTIALS OF DIAGNOSIS
Decreased FEV1 and FVC are characteristic findings.
DLCO is also decreased; however, the hallmark of restrictive disease is decreased TLC.
Etiology includes musculoskeletal and neurologic disorders.
Restrictive lung disease can be grouped into three main categories based on etiology: intrinsic lung disease, chest wall abnormalities, and neuromuscular disease. All are associated with a restrictive pattern of disease that leads to reduced FEV1 and FVC (with a normal or elevated ratio) and decreased DLCO; however, the hallmark of this condition is decreased TLC. The TLC value is used to classify restrictive lung disease as mild, moderate, or severe. Mild disease is indicated by a TLC that is 65–80% of the predicted value; moderate, by a TLC that is 50–65% of the predicted value; and severe, by a TLC that is less than 50% of the predicted value.
Among the numerous causes of intrinsic lung diseases are asbestosis, sarcoidosis, berylliosis, and pulmonary fibrosis. The inflammatory response associated with these disorders leads to V/Q mismatching that predominantly affects perfusion, thereby promoting shunt physiology. Patients with these disorders are often tachypneic, with a compensatory decreased partial pressure of carbon dioxide (Pco2). Chest wall abnormalities encompass a range of conditions that inhibit full expansion of the chest wall; these include spinal curvature disorders (eg, kyphoscoliosis), thoracic cage problems, and severe obesity. Neuromuscular diseases, such as polymyositis, myasthenia gravis, and muscular dystrophy, cause diffuse muscle weakness, decreased TLC, and a subsequent restrictive lung disease pattern.
ESSENTIALS OF DIAGNOSIS
Low FEV1/FVC ratio, with values at or below the fifth percentile of the lower limit of normal.
DLCO and TLC aid in diagnosis of specific disorders: DLCO is low and TLC increased in patients with emphysema, whereas DLCO is normal in chronic bronchitis and preserved or elevated in asthma.
Obstructive lung diseases are identified by FEV1/FVC ratios that are below predicted values. Formerly, an obstructive disease was diagnosed when these ratios were 70% of the predicted values or lower. However, because these ratios decline with age, using this method can result in an overestimate of the prevalence of these diseases. Consequently, using the fifth percentile of the lower limit of normal has been suggested as a more accurate method of defining obstructive lung diseases.
In contrast to restrictive lung disease, where disease severity is classified using TLC, obstructive lung disease severity is differentiated according to the FEV1. Mild obstruction is defined as an FEV1 that is 65–80% of the predicted value, moderate as 50–64% of the predicted value, and severe as less than 50% of the predicted value. Additionally, TLC and DCLO values help to identify the specific obstructive lung disease causing a patient’s symptoms, for example, emphysema, chronic bronchitis, or asthma.
Emphysema is defined by the destruction of airways distal to the terminal bronchioles without evidence of fibrosis. In patients with emphysema, DLCO is decreased, TLC is increased, and symptoms are not reversible with bronchodilators. Emphysema can be further differentiated radiographically into proximal acinar, panacinar, and distal acinar disease. Proximal acinar (or centriacinar) disease is seen predominantly in the upper lobes and causes cystic lesions. Panacinar destruction is often seen in patients with α1-antitrypsin deficiency. Paraseptal emphysema often leads to bullae that cause spontaneous pneumothoraces.
Chronic bronchitis is defined on the spectrum of COPD by the presence of a chronic cough that occurs at least 3 months of the year for 2 consecutive years. In contrast to emphysema, there is minimal destruction of distal airways, which preserves the DLCO as normal. However, like emphysema, symptoms in chronic bronchitis are irreversible with bronchodilators.
In patients with asthma, the FEV1/FVC ratio is usually less than 70% of the predicted value or below the fifth percentile of the lower limit of normal. Bronchoconstriction and symptoms of impaired lung function occur during episodic attacks, but patients may seem unaffected between acute episodes. In order to induce findings characteristic of the underlying disease, a methacholine challenge test may be performed. This involves administering a potent bronchoconstrictor through a nebulizer to the patient, which activates the muscarinic M3 receptor, thereby inducing bronchoconstriction. Spirometry can then be performed to analyze the FEV1/FVC ratio. A bronchodilator is often given afterward to demonstrate reversibility. In asthmatic patients, the DLCO is preserved and sometimes even elevated.
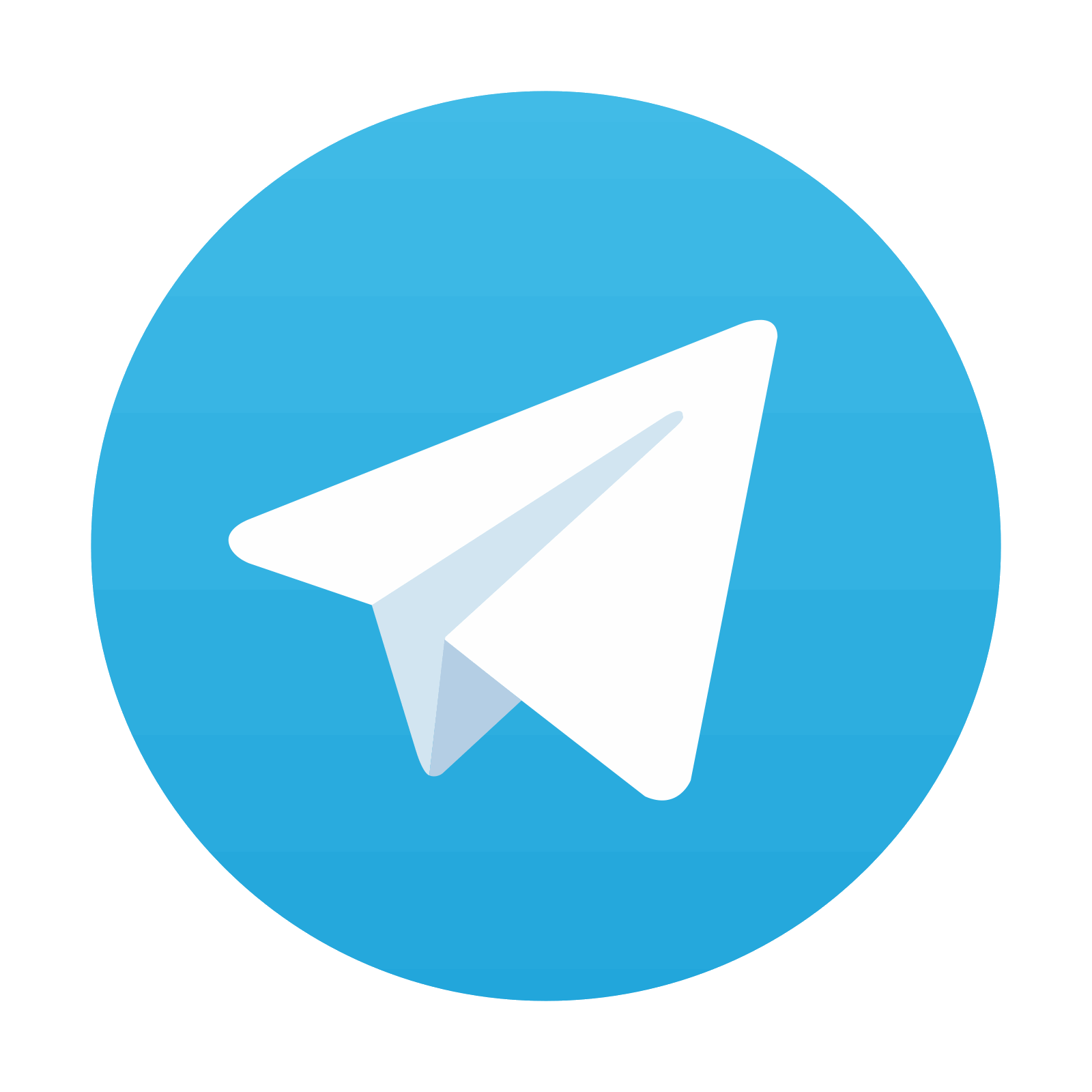
Stay updated, free articles. Join our Telegram channel
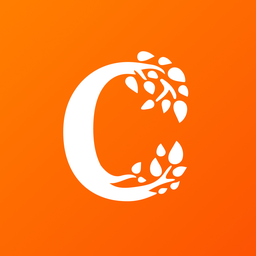
Full access? Get Clinical Tree
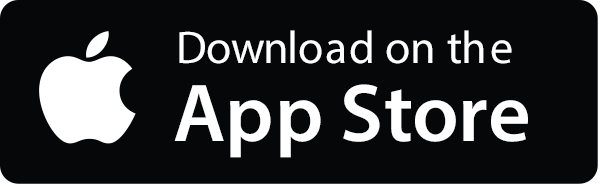
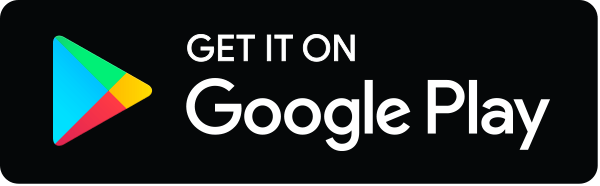
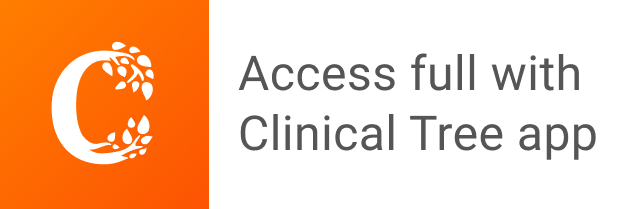