Fig. 3.1
Cross section of a nerve fiber demonstrating axons, fascicles along with the surrounding connective tissue layers: endoneurium, perineurium, and epineurium (Reprinted with permission from the Cleveland Clinic Center for Medical Art & Photograph © 2009–2014. All Rights Reserved)
In developing an understanding regarding the classification of nerve injury, one must pay homage to both H. J. Seddon and Sir Sydney Sutherland. In a landmark paper (1943) based on the patterns of injury sustained by soldiers during World War II, Seddon classified nerve injury into three types: neurapraxia, axonotmesis, and neurotmesis. Later, in 1951, Sunderland subcategorized neurotmesis into three separate types [8]. While the two classification schemes are often confused, they are complementary in that both base their taxonomies on damage to the nerve’s anatomic layers (Table 3.1, Fig. 3.2).

Table 3.1
Classifications and descriptions of nerve injury
Seddon classification | Sunderland classification | Description of injury |
---|---|---|
Neurapraxia | Grade I | Segmental demyelination without axonal injury |
Axonotmesis | Grade II | Loss of continuity of axon without damage to outer layers (endoneurium, perineurium, epineurium) |
Neurotmesis | Grade III | Loss of continuity of axon and endoneurium with intact perineurium and epineurium |
Grade IV | Loss of continuity of axon, endoneurium, and perineurium with intact epineurium | |
Grade V | Loss of continuity of the entire nerve trunk |

Fig. 3.2
Diagrammatic representation of the Sunderland classification of nerve injury. Each of the five categories of nerve injury demonstrates an increasing level of nerve fiber damage. Severity of damage corresponds to the layers of connective tissue that have been injured (Used with permission from Sunderland [8])
Neuropraxia (Sunderland Type I)
This category of nerve injury involves a brief, focal period of stress, ischemia, radiation, or electrical injury to the nerve, which results in conduction properties with or without changes in myelin structure. The resulting conduction block also leads to changes in motor recruitment properties of the target muscle. While conduction of signals originating proximal to the lesion is attenuated, signals originating distal to the lesion do not show any attenuation. These attenuation properties of the signal can help localize the lesion using electrodiagnostic testing. Nerve recovery is generally complete by 12 weeks (Fig. 3.2) [4].
Axonotmesis (Sunderland Type 2)
This category of nerve injury results in damage to the axon but leave the basal lamina, endoneurium, and all the outer layers of the nerve intact. The axonal damage is followed by Wallerian degeneration of the distal axonal stump and regeneration of the proximal axonal stump [9, 10]. Motor end plates degenerate by 12–18 months following injury after which the muscle cannot be reinnervated and muscle atrophy is irreversible. Since axons regenerate at rate of 1–3 mm/day, the proximity of a lesion to the motor end plate is predictive of recovery (Fig. 3.2) [11].
Neurotmesis (Sunderland Types 3, 4, and 5)
In type 3 nerve injury, the endoneurium is damaged along with the axon, but the perineurium and epineurium are intact. Type 4 lesions involve damage to the endoneurium and perineurium, but the epineurium and the continuity of the nerve itself is not interrupted. Type 5 lesions involve the epineurium and can disrupt the continuity of the nerve. Type 4 and 5 lesions can lead to the development of significant scar tissue that will impede regeneration. Successful regeneration may require debridement of scar tissue and microsurgical repair of the nerve segments [12]. The distal nerve stumps in type 2–5 lesions continue to conduct for at least 1 week. Compound muscle action potentials can be elicited for 7 days, whereas nerve action potentials can be elicited for up to 10 days. Electrodiagnostic testing during this time period can be useful finding the location of the lesion [4]. Mackinnon introduced a type 6 injury classification, which is defined as nerve injury that comprises two additions to the previously discussed subtypes. Specific to amputation and replantation, all nerve injuries are categorized as neurotmesis (Sunderland Type 5) (Fig. 3.2).
Wallerian Degeneration and Axonal Regeneration
A full review of Wallerian degeneration and the biochemical events accompanying axonal regeneration is not within the intended scope of this chapter and is readily available elsewhere [13, 14]. Nevertheless, several key events should be highlighted. The first is that following axonal disruption, Wallerian degeneration (retrograde axonal degeneration) within the distal and proximal stumps and axonal regeneration of the proximal stump begins within a few hours. Retrograde axonal degeneration of the proximal stump stops at the first proximal internode in mild injuries but may continue for a few centimeters proximally in more major injuries [15]. This becomes important in the assessment of nerve regeneration because the “regeneration front” actually occurs proximal to the site of repair. The second important concept is that the transection of a peripheral nerve heralds a panoply of events which contribute to both axonal degradation (Wallerian degeneration) and axonal regeneration. Wallerian degeneration begins with the prompt buildup of intracellular calcium and the activation of calpains and phospholipases necessary for cytoskeletal degradation within the proximal and distal stumps [4, 14, 16–18].
Wallerian degeneration and the acute inflammatory events that accompany it set the stage for axonal regeneration, which is heralded by the remarkable transformation of Schwann cells from supportive cells to proliferative cells. This transformation includes the decreased production of normal Schwann cell proteins (PMP22, P0, and connexin-32) and the production of axonal growth factors (GAP-43, neuregulin) [19, 20].
Transection of the peripheral nerve leads to chromatolytic changes within the cell that ultimately indicate a switch in the cellular process program from a transmitting mode to a growth mode. Within the next 12 h, there is an increased production of neutrophils, growth-associated proteins (GAP-43), insulin-like growth factors (IGFs), and glial-cell-derived neurotrophic factors (GDNFs), as well as a plethora of other proteins that promote axonal sprouting, growth cone development, and neurite formation [21, 22].
Extracellular matrix proteins (laminin and fibronectin) produced by Schwann cells form the connective tissue pathways between the nerve stumps. Schwann cells migrate along these pathways and form bands of Bungner. As proximal stump axons begin to regenerate, Schwann cells within the distal nerve stump secrete growth factors (BDNF, GDNF), which serve as a chemoattractant gradient guide for the growth cones. Topographical cues provided by the specific cell adhesion molecules present on the connective tissue pathways aid in growth cone attachment and migration [13]. When Schwann cells are contacted by these regenerating axons, they alter their phenotype and begin producing myelin to encase the newly growing axon.
The greatest obstacles for the regenerating axons include bridging a gap between the proximal and distal segments and overcoming the presence of scar tissue. Even if axons reach the endoneurial tubes of the distal stump, functional recovery is dependent on whether the axons can reach the motor end plates to reestablish functional neuromuscular junctions before the end plates degenerate. Hence, in replantation the reduction (or avoidance) of intraneural scar tissue is of critical importance. Motor end plates begin to degenerate at 18–24 months. Whole muscle fibrosis can begin as early as a few weeks after injury. Schwann cells maintain their ability to promote regeneration for about 18 months. After this time period, they lose their ability to promote regeneration but are still able to myelinate growing axons. Sprouting axons attempt regeneration for up to 12 months following injury [4].
Types of Nerve Repair
Throughout the long and storied history of replantation and nerve repair, one principle which has stood the test of time above all others is the concept of tension free repair as championed by Millesi [23]. The inflammatory response induced by Wallerian degeneration, the cocktails of cytokines and growth factors produced by Schwann cells, and the advanced methods of rehabilitation are of minimal importance if peripheral nerves are repaired under tension. Specific techniques of peripheral nerve repair are based on the nature of injury and the condition of the nerve as well as on the size of the nerve (e.g., sciatic vs. digital). Amputations can result in avulsion injuries, crushing injuries, or clean guillotine-like lacerations. Regarding operative sequence, it is our preference in digital replantation to repair the nerve after the digit has been revascularized and before the venous anastomosis.
Epineural Repair
If the injury is a clean-cut injury without contusion, the standard of care is to perform an end-to-end epineural repair (Fig. 3.3). The first goal of the epineural repair is coaptation of the nerves without tension [24, 25]. Because the bone has often been shortened to facilitate replantation, primary repair without tension is usually not difficult. The second goal is to reduce the formation of regeneration inducing scar tissue (see above). This is achieved by the epineural placement of sutures, performing minimal intraneural dissection, and using as few stitches as necessary to perform the repair. While the number of sutures needed to coapt a nerve is far smaller than what is needed, for example, in the arterial repair, it depends partly on the nerve being repaired. Four to eight simple interrupted sutures are used to approximate the two ends [12]. For digital nerves, usually three stitches are adequate and for larger nerves more stitches might be necessary. Nerve repair should be performed using an operative microscope for two reasons. First, the operative microscope aids in carefully placing stitches in the epineurium only, thus reducing the internal scar tissue which impedes regeneration, and secondly, magnification significantly aids in identifying the superficial vessels that are visible along the longitudinal aspects of the nerve segments (vasa nervorum). Aligning these vessels is an excellent method for the alignment of the fascicles, which significantly reduces misdirected axonal regeneration [26]. While it is not our preferred method, some groups have successfully performed sutureless epineural repair using fibrin glue [27].


Fig. 3.3
Epineural repair of nerve with sutures passing only through external and internal epineurium and without violation of the perineurium (Reprinted with permission from the Cleveland Clinic Center for Medical Art & Photograph © 2009–2014. All Rights Reserved)
Epineural Sleeve Repair
Epineural sleeve repair is a variation of epineural repair that is used when there is some contusion associated with the tips of the transected nerve stump (Fig. 3.4). The repair calls for peeling back the epineural sheath on the distal nerve stump and trimming back the contused fascicles by 2 mm. The fascicles are aligned using cues from the vasa nervorum, and the distal epineural sleeve is then sutured to the proximal sleeve. This repair is potentially advantageous compared to standard epineural repair, because it moves the suture lines away from the regenerating fascicular ends. There are no clinical comparisons that have proven significant statistical advantage of this variation to standard epineural repair methods [26].


Fig. 3.4
Epineural sleeve repair requires peeling back the epineurium and trimming back of the contused fascicles followed by placement of epineural sutures away from the location of the regenerating fascicles (Reprinted with permission from the Cleveland Clinic Center for Medical Art & Photograph © 2009–2014. All Rights Reserved)
Fascicular Repair
Nerve ends can also be coapted using fascicular or grouped fascicular repair (Fig. 3.5). In this case, the individual fascicles are aligned using perineural sutures, or groups of fascicles are aligned using sutures within the internal epineurium. Even though the technique provides superior fascicular alignment, it requires the use of at least two sutures per fascicle, which causes intraneural scar development and the reduction of blood flow that can worsen the final result. The method has fallen out of favor because clinical studies show no advantages of this technique over epineural suturing [26]. For nerves that are partially injured, however, there might still be a role for limited fascicular repair.


Fig. 3.5
Fascicular repair or grouped fascicular repair involves aligning the fascicles by placing sutures in the perineurium (Reprinted with permission from the Cleveland Clinic Center for Medical Art & Photograph © 2009–2014. All Rights Reserved)
End-to-Side Repair
If one of the severed nerve segments is not available for coaptation, an end-to-side repair can be performed. The evidence on the overall clinical outcome is highly variable. Some of the variability in the outcome is related to the lack of a clear understanding of how axons from the donor nerve pass into distal segment, thus begging the need for further inquiry both in the laboratory and in the clinical setting [28]. Recent evidence comparing end-to-side repair with conventional (end-to-end) repair techniques indicates that end-to-side repair have functionally inferior outcome and should be used only as salvage procedures [29].
Nerve Conduits
Despite the bony shortening that often accompanies extremity or digital replantation, there are several scenarios where Millesi’s critical tenet of tensionless repair is not possible. These include injuries involving segmental loss of the nerve, crushing injuries, and nerve avulsions. Also, when the nerve cannot be repaired primarily, there is significant contraction, making direct end-to-end tensionless repair unfeasible. While not typically a problem in the acute repair setting of a replant, the proximal and distal nerve stumps in clean-cut lacerations can contract by 4 % in the first week, 8 % in 3 weeks, and up to 28 % if left unrepaired over an extended time period [30]. Direct repair of contracted nerve segments place a high level of tension on the nerve. The increased tension leads to nerve ischemia and ultimately fibrosis of the nerve segment [26].
Nerve Autografts
When direct repair of a transected nerve is not possible, a nerve graft, nerve conduit, or nerve transfer needs to be considered in order to achieve functional restoration. The gold standard of reconstructing a nerve gap is with a nerve autograft. Autografts provide resident Schwann cells as well as the microarchitecture necessary to guide sprouting axons. Some of the candidates for autograft harvest include sural nerve (30–50 cm), dorsal cutaneous branch of the ulnar nerve (4–6 cm), superficial branch of the radial nerve (20–30 cm), lateral antebrachial cutaneous nerve (10–12 cm), and the medial antebrachial cutaneous nerve (10–12 cm). Autograft harvest, while imperative in some cases, should be used cautiously as it can lead to significant donor site morbidity, which includes hypesthesia at the donor site, increased neuroma formation in the donor nerve, and (usually) a separate incision [12].
It is our preference to use the sural nerve for autograft as it is a relatively easy harvest site, can be harvested by a separate team while the recipient site is being prepared, and results in relatively minimal donor site morbidity. On the other end of the spectrum, using the sensory branch of the radial nerve should be avoided at all costs as numbness and parasthesias associated with its harvest can be devastating to the patient. In selecting a nerve for autografting, several principles should be followed. First, size is important. Larger grafts can lead to central neural necrosis, and increased scar tissue formation, which can impede regeneration. Secondly, sensory nerve autografts containing small diameter fibers can lead to mediocre functional outcome due to the mismatch of size. Thirdly, a risk-benefit analysis should be performed for each donor site and the one with the least morbidity should be selected. Since there are limited options for autografts, research has been carried out to better understand the potential of biological and artificial conduits to fill nerve defects [31].
Biological Conduit Repair
Given the limited options of nerve autografts as well as the well-known morbidity in using them, multiple candidate materials for biological nerve conduits have been explored including arteries, veins, skeletal muscle, and epineurium. Veins have also been successfully used as inside-out or turn-over grafts. The collagen, laminin, and Schwann cells, which are found on the adventitia of veins, contribute to the increased speed of axonal regeneration found in turn-over vein grafts when compared to standard vein grafts [32]. Combination biological grafts containing vein, filled with skeletal muscle, have been found to be useful in filling nerve gaps ranging from 0.5 to 6 cm. Skeletal muscle tissue contains longitudinally oriented basal lamina which facilitates contact adhesion of migrating Schwann cells and ingrowing axonal fibers. Additionally filling the vein with muscle prevents the graft from collapsing [31]. Tendon grafts, which have similar longitudinal microarchitecture, have also been shown to be effective nerve conduits [33]. Epineural grafts are derived from neural tissue and produce large amounts of laminin B2 and VEGF, which augments Schwann cell attachment and axonal growth. Furthermore, epineural grafts are less immunogenic than the other biological conduit materials and could therefore even serve as an effective allograft. [26] It is our preference, when there is a limitation of nerve autograft or when the risks of harvest outweighs the benefits, to use turn-over vein grafts as they are relatively easy to harvest, are in abundant supply, and can usually easily be found in the same surgical field as the nerve that is being repaired (e.g., in forearm for cases of digital nerve reconstruction).
Artificial Conduits
Artificial nerve conduits were initially made of flexible, non-immunogenic but nonbiodegradable materials such as silicone and PTFE. Silicone conduits tend to develop a capsule of circumferentially oriented smooth muscle myofibroblasts, which constricts the conduit and the growing nerve segment [34]. Morbidities caused by the scarring and constriction of ten requires reoperation for removal of these conduits. Furthermore, silicone is nonpermeable and does not allow the exchange of larger biomolecules that may be beneficial to the growing axon [15]. Given the limitation of these early artificial conduits, newer conduit materials have been utilized including synthetic biodegradable materials such as aliphatic polyesters and polyphosphoesters as well as naturally occurring biodegradable materials such as collagen, hyaluronic acid, and fibrin. Aliphatic polyesters include various biodegradable non-immunogenic polymers such as polyglycolic acid (PGA), poly-(lactic acid) (PLA), polycaprolactone (PCL), poly-lactide-coglycolide (PLGA) copolymer, poly-(L-lactic acid) (PLLA), and poly-(3-hydroxybutyric acid) (PHB) [15]. PGA is a commonly used material in sutures and mesh. It is broken down by hydrolysis within 90 days. PGA tubes are commercially marketed to bridge sensory nerve gaps less than 30 mm (Neurotube, Synovis Life Technologies Inc., USA). The copolymerization process used to synthesize aliphatic polyesters such as PLGA and PLLA can be altered to vary degradation times, thermal properties, mechanical performance, and wettability of these materials. The permeability of these materials which is important to maintain the nutrient exchange and as well as growth factor leakage from the conduits can also be adjusted by adjusting the crystallinity of these synthetic materials [31].
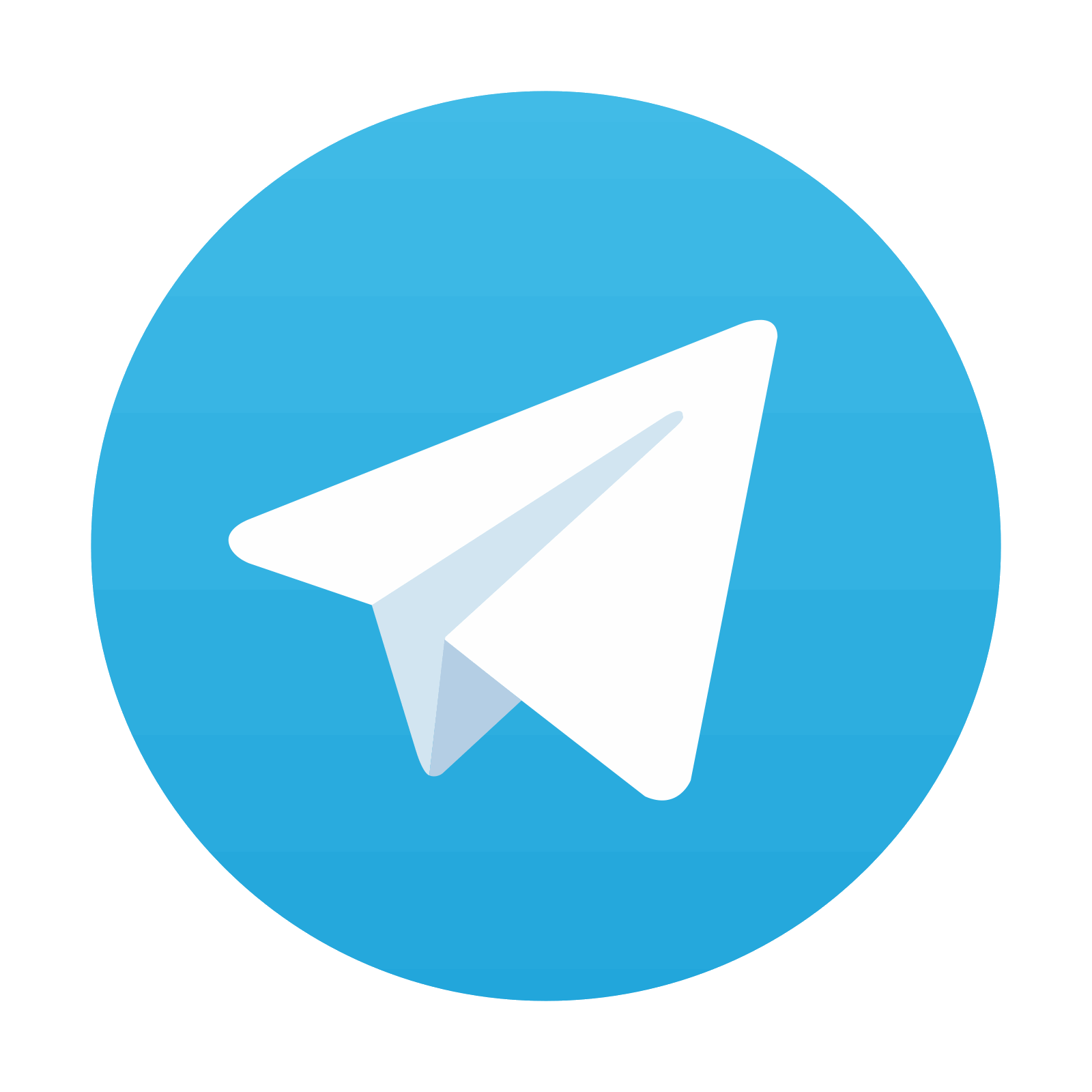
Stay updated, free articles. Join our Telegram channel
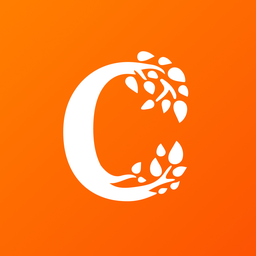
Full access? Get Clinical Tree
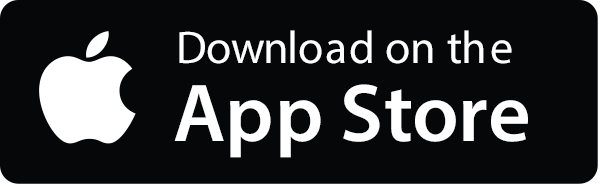
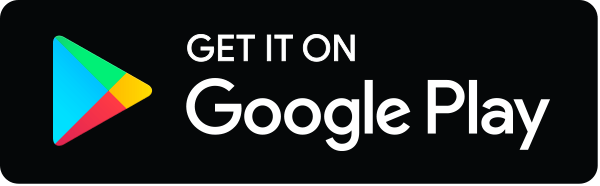