Fig. 2.1
Leonardo da Vinci: The proportions of the human figure (Vitruvian Man) (circa 1490). (Original at: Gabinetto dei disegni e stampe of the Gallerie dell’Accademia, Venice. In Public Domain (Photo from: http://en.wikipedia.org/wiki/File:Vitruvian.jpg In Public Domain))
Bone
Anatomy
Bone is a unique, well-organized, and dynamic tissue. Its composition and intricate structure make it resilient to stress while at the same time lightweight, making it ideal for mechanical support, motion, and grasp. It serves as attachment points for muscles, tendons, and ligaments and as a reservoir for calcium hemostasis. At the macroscopic level, there are two major forms of bone: cortical and cancellous. Cortical bone, also known as compact bone, comprises approximately 80 % of the skeleton and forms the cortex, or outer shell, of most bones. It is four times denser than cancellous bone [1]. The strength of cortical bone is due to its intricate architecture with the fundamental unit being the haversian system or osteon. The osteon is a cylindrical structure several millimeters long running parallel to the long axis of the bone. At the core of the osteon is a tubelike structure called the central or haversian canal, which houses capillaries and poorly myelinated nerve fibers. Surrounding the haversian canal are concentric rings called lamellae. In between lamellae are osteocytes, former osteoblasts now surrounded by a bone matrix and housed in a space termed lacunae. Branching from each lacunae and running approximately tangential to each lamellae are small channels called canaliculi. It is through these small channels that osteocytes send out extensive cell processes thereby establishing contact with nearby osteocytes.
Cancellous bone, also known as trabecular bone, has approximately one fourth the mass of cortical bone. It is softer, weaker, and more elastic. This is due in part to its structure consisting of branching bony struts or spicules that are organized into a loose network typically aligned along areas of stress. Relatively large spaces exist in between struts making cancellous bone quite porous. This gives it a larger surface area to volume ratio. Because bony turnover is proportional to the surface area available, cancellous bone has approximately eight times greater metabolic turnover than cortical bone.
At the microscopic level, there are two major forms of bone: woven and lamellar. Woven bone, also called primary bone, is the bone that is formed de novo, making it the initial tissue in bone formation. It can be either immature, as seen in the embryo and with fracture callus formation, or pathologic and formed by bony tumors. Woven bone is not stress oriented and is weaker and more flexible compared to lamellar bone.
Lamellar bone, considered normal bone, is formed after the remodeling of woven bone and can be either cortical or cancellous. It is highly organized and forms more slowly than woven bone.
Bone Healing
Unlike many other tissues of the body that form scar tissue during the healing process, fracture healing restores injured bone to its original state with the same biological and mechanical properties it once had. The main factors contributing to proper healing of bone include an adequate blood supply, progenitor cells, growth factors, and an extracellular matrix.
Bone blood flow is the major determinant to how well a fracture heals for it carries nutrients, oxygen, and other essentials to the injury site. Grossly, vessels damaged at or near the site of bone injury need to be repaired in order to provide adequate blood supply. At the microscopic level, angiogenesis takes place within the periosteal tissues and marrow space in order to provide blood flow to the fracture site. The importance of the periosteal circulation for healing leads directly to the recommendation of minimal bone stripping when performing osteosynthesis during replantation.
Bone healing also requires the recruitment of progenitor cells. This occurs as mesenchymal stem cells are brought to the fracture site, both from surrounding soft tissue as well as from the systemic circulation [2]. These cells in turn differentiate into osteoblasts, osteoclasts, chondrocytes, and fibroblasts. The recruitment and differentiation of mesenchymal stem cells is dependent on growth factors present at the site of injury.
The extracellular matrix (ECM) provides the scaffolding for new bone formation. Collagen is the major component of the ECM. Types I and IV make up the majority of bone, where types II, IX, X, and XI make up the majority of cartilage. In addition to collagen, glycoproteins and proteoglycans also make up the ECM.
The repair process of bone can be classified into two major forms of healing: primary and secondary. The type of healing that occurs is determined by the means of fracture fixation and the strain (Δlength/original length) experienced across the fracture. Primary (direct) bone healing occurs predominantly via intramembranous ossification. This occurs when rigid fixation and absolute stability is achieved with the two ends of bone in contact with one another. With minimal motion or gapping at the fracture site, strain is typically less than 2 % and an intermediate cartilaginous callus does not form [3]. Instead, the haversian system of remodeling takes place; osteoclasts cross the fracture site via cutting cones, and osteoblasts occupy the canal left in their wake to later organize and become osteons. The two ends of the fracture are joined by lamellar bone.
Secondary (indirect) bone healing is the predominant form of fracture healing. It occurs primarily via enchondral ossification though in some cases intramembranous healing also plays a role [4]. Secondary or enchondral ossification refers to fracture healing that occurs via a cartilage callus intermediate. This callus develops because of greater motion and less stability at the fracture site resulting in greater strain, typically between 2 and 10 % [3]. This creates an environment optimal for undifferentiated mesenchymal cells to become chondrocytes and establish a soft callus. The amount of callus that develops is inversely proportional to the degree of fracture immobilization that is achieved. Angiogenesis occurs within the fracture callus, and over time as greater stability is achieved, chondrocytes undergo apoptosis and are replaced by osteocytes. Woven bone is initially formed within the soft callus transforming it into a hard callus. This later undergoes remodeling as woven bone becomes lamellar bone in response to mechanical stress. If the strain at the fracture site is too great, however, an environment is created unsuitable for osteocytes or chondrocytes [5]. Instead, fibroblasts, the initial cell type to populate the fracture site, persist and a fibrous nonunion develops.
Principles of Fracture Fixation
In the setting of replantation, fracture fixation is paramount in order to provide stability and protect the vascular repair. Different means of fixation create varying degrees of stability. As mentioned above, stability influences which type of bone healing, if any at all, will occur. In the upper extremity and the hand, the most common types of fixation include wire sutures, Kirschner wires, lag screws, plates, and external fixators. Fracture location and pattern, soft tissue status, wound contamination, and surgeon preference all influence the type of fixation used. Furthermore, osteosynthesis techniques useful in the acute setting differ from those employed for secondary or revisional surgery.
One of the most useful techniques for fixation in the setting of both replantation and secondary procedures, such as toe-to-hand transfer, is intraosseous wiring [6, 7]. While not as rigid as plate or lag screw fixation, intraosseous wiring has been successfully used for fracture fixation in the hand and for small joint arthrodesis for over 35 years. Union rates for fractures treated with this technique are uniformly high, ranging from roughly 98.5–100 % [8–10]. The technique has the additional advantage of being applicable to very short (5 mm) bone segments such as may occur when amputations occur proximate to joints. In addition, the technique requires only minimal dissection in order to expose adequate bone surfaces, thus minimizing interference with blood supply to the bone via the periosteum.
Preparation for intraosseous wiring begins with shaping of the surfaces to be joined. In most cases, this will involve leveling of the proximal and distal segments so that the union line approximates a purely transverse fracture. The raw surfaces of the cortical bone should be as smooth and congruent as possible so as to maximize contact area and minimize motion following synthesis. Next, the soft tissues and periosteum are dissected minimally away from the free surfaces; the exposed bone should allow for 2 mm of distance between the intraosseous wires and the union site. Beginning with either the proximal bone stump or the distal, amputated segment, two holes are drilled utilizing either a 1 mm drill bit or a 0.045 in. diameter K-wire. Configuration of these bicortical tunnels may be either parallel or in a 90–90 (perpendicular) configuration (Fig. 2.2a–d); both have demonstrated equal union rates. The remaining segment is then aligned against the previously prepared bone fragment, and corrections for any malrotation are made. Tunnels are then made parallel to those in the original segment. 2-0 (28 gauge) stainless steel wires may then be passed through the tunnels. While one member of the team holds the bone segments in proper alignment, a second operator twists the free wire ends to tighten the loops. Final check of alignment should be confirmed prior to completely tightening the wires, as small adjustments in rotation may still be made. After trimming the wires, the free ends should be rotated down adjacent to the union site and away from any tendons.


Fig. 2.2
(a–d) Diagrams of different intraosseous wire techniques. (a) In the parallel technique, holes are drilled equidistant from the fracture site and parallel to those in the opposite segment. (b) Wires are passed and twisted. (c) In the 90–90 technique, holes on one side are drilled perpendicular to one another with mirror image holes drilled in the opposite segment. (d) Wires are passed between holes in the same plane
Osteosynthesis via intraosseous wiring does provide adequate rigidity for early rehabilitation, and, since wires transgress neither tendon nor skin, the technique provides little resistance to the gliding of surrounding soft tissues. Finally, the resources necessary for this technique (k-wires and stainless steel wire) are inexpensive and universally available. Limitations are primarily in the setting of amputation where the fracture line is long and oblique and where conversion to a transverse pattern would result in excessive shortening. In this setting, a single intraosseous wire may be combined with one or more K-wires.
Kirschner wires (K-wires) are a widely used fixation method, both temporarily prior to other means of fixation, and permanently until bony union occurs. Advantages of K-wire fixation include low cost, means of percutaneous application thus limiting soft tissue injury, speed of application limiting warm ischemia time, and ability to be easily removed once bony union occurs.
The degree of stability offered by K-wires is influenced by the pin diameter and configuration. For transverse phalanx fracture patterns, crossed wires offer the greatest stability to torsion, distraction, and bending [11]. For oblique fracture patterns, pins oriented perpendicular to the fracture line offer greater stability to torsion, distraction, and bending over crossed pins [11]. With regard to compression, however, pins oriented perpendicular to the shaft of the bone offer greater stability [12]. For oblique fracture patterns, therefore, the application of multiple wires in different orientations provides the greatest stability. In the setting of significant bone loss not allowing for anatomic reduction, an intramedullary pin inserted prior to neurovascular repair allows for rotational adjustment and realignment of the vessels prior to additional wire placement [13].
There are disadvantages to K-wire fixation, however. Percutaneous wires have a significantly increased risk of pin tract infection [14–16], which may prompt early removal of hardware, potentially leading to instability at the fracture line. By nature of their placement, K-wires do not compress the fracture site; instead, they offer relative stability thus increasing the risk of nonunion and malunion compared to other means of more rigid fixation [17]. Finally, as noted above, K-wires may impede motion of soft tissue, including tendons, during rehabilitation.
An alternative to the intraosseous wire/oblique K-wire technique for longer oblique fracture lines is to join the bone segments via lag screws. While providing some of the best rigidity and compression of any fixation method, the injury must meet specific criteria for lag screws to be applicable. The amputation line must be oblique enough to accommodate placement of at least two screws without encroaching on the free edge of the fracture line. Bone loss should be minimal so that good contact of cortical surfaces can be achieved nearly, if not completely, circumferentially. Finally, no comminution at the fracture site should be present. Lag screw application starts with the use of pointed reduction clamps to maintain reduction while aligning the drill perpendicular to the fracture line. The near cortex is drilled to match the outer thread diameter of the screw thereby creating a gliding hole. A drill sleeve or guide is placed into the hole, thus insuring both stages of drilling are in line. The far cortex is then drilled with the appropriately sized drill bit, typically to match the screw’s core size, creating a thread hole. For example, placement of a 1.0 mm screw would require a 1.0 mm drill bit to create the gliding hole and a 0.8 mm drill bit to create the thread hole. Countersinking, or tapping of the outer surface of the near cortex to match the screw head size, is typically performed to decrease the amount of screw head protrusion and reduce surrounding soft tissue irritation.
The number of screws used is dependent on the length of the fracture line. A fracture line whose length is more than twice the diameter of the diaphysis typically requires two or more screws (Fig. 2.3a–b). When a screw is inserted close to the apex of a fragment, ensuring that the distance from the fracture line to the edge of the screw is no less than the diameter of the screw head will decrease the risk of fracturing off the fragment apex.


Fig. 2.3
(a, b) Lag screw fixation. After drilling the gliding hole into the near cortex, a drill guide is inserted prior to drilling the thread hole into the far cortex (a). (b) Final screw placement with countersinking of the gliding hole demonstrates how screw head protrusion can be minimized
Open reduction and internal fixation with the use of miniplates and screws offers one of the most broadly applicable and stable forms of osteosynthesis for complex fractures in the small bones of the hand. At the level of the forearm or arm, rigid plate fixation, often with a dynamic compression technique, would be the standard for repair of the bony injury in the setting of amputation (see Chap. 7). Advantages also include adequate stability for early motion and rehabilitation [18–21]. Despite the versatility of the technique, it is rarely employed in the setting of primary replantation at the level distal to the wrist, largely because plate application requires extensive soft tissue dissection, potentially jeopardizing local blood supply to the union site, and longer operative time in what will already be a lengthy procedure. More commonly, plate and screw osteosynthesis will be used in the setting of secondary surgery for nonunion or malunion (see Chaps. 13 and 14).
While the majority of successful replantations will proceed to bony union, nonunions may still occur. Incidence of nonunion varies depending on the primary osteosynthesis technique employed, ranging from as low as 1.5 % with intraosseous wiring [10] to 9–19 % with K-wires alone [17]. Nonunions are defined by the lack of the potential to heal without further intervention and can be classified as either atrophic or hypertrophic. Atrophic nonunions lack any callus and are physiologically defined by a poor blood supply to the fracture site. Successful healing of an atrophic nonunion requires the use of cancellous bone grafts in addition to plate fixation. Cancellous bone graft is osteoconductive, acting as a structural framework or scaffold for new bone growth [22, 23]. If an autograft is used, harvested from the patient, and transferred at the time of surgery, the graft is also said to be osteoinductive, containing growth factors that stimulate osteoprogenitor cells to differentiate into osteoblasts during the process of new bone formation. Compared with cortical bone grafts, cancellous grafts heal more rapidly and are more resistant to infection [24].
Hypertrophic nonunions have an adequate blood supply to the fracture ends and an abundance of callus formation. They occur due to poor stability and excessive motion at the fracture site. In this setting, secondary surgery typically addresses take down of the fibrous nonunion and plate fixation with or without the use of a bone graft.
Successful bone healing, albeit in a malrotated or angulated position, occurs in anywhere between 2 and 14 % of replanted digits [17]. When faced with malunion and unacceptable bony alignment, secondary surgery requires a corrective osteotomy (see Chap. 14). In cases where significant bone loss occurred at the time of amputation, or if significant bone needs to be removed in order to correct alignment, corticocancellous grafts can be applied. Cancellous bone grafts alone are not enough to provide structural support and maintain alignment after correction is made [25]. Cortical bone, either autograft or allograft, is tailored and shaped to fill the bony void so that continuous cortical bone exists circumferentially around the site of correction. Typically, a plate is applied over the bone graft to help maintain its positioning and decrease the risk of shifting of the graft before it is fully incorporated.
Tendon
Tendon Anatomy
Knowledge of tendon anatomy is paramount to understanding tendon homeostasis and to performing successful tendon repair in the setting of replantation. Tendons play an essential role in the musculoskeletal system, transmitting tensile loads from muscle to bone, resulting in joint stabilization and movement. The primary cell type in tendon is the tenocyte. These fibroblast-like cells function to produce collagen and other extracellular matrix proteins. Type I collagen accounts for approximately 70 % of tendon composition. Other types of collagen (III, V, IX, X, XI, and XII) are present in lesser amounts and contribute to overall tendon homeostasis [26]. Tendons are sequentially composed of collagen molecules, fibrils, and fascicles arranged into parallel primary, secondary, and tertiary bundles. Each bundle is surrounded by an endotenon that contains both blood vessels and nerves [27]. An epitenon and paratenon layer surrounds the tendon unit with a thin layer of synovial fluid in between and function in tendon regeneration, repair, and gliding.
Tendons are well-vascularized structures with a distinctly different blood supply depending on the presence or absence of a synovial sheath. Extensor tendons and flexor tendons proximal to the A1 pulley of the hand receive their blood supply by way of segmental branches from nearby arteries coursing through adjacent connective tissue. Blood vessels enter the tendon unit through the para- and epitenon, forming a vascular network around each fibril to provide nutrition to individual bundles via the endotenon [28].
Digital flexor tendons distal to the A1 pulley are unique in that a synovial sheath is the source of nutrition. The flexor sheath is composed of a fibrous portion and a synovial portion with both visceral and parietal layers. Its primary mode of providing tendon nutrition is through a combination of local diffusion and direct vascular perfusion via specialized mesotenon called vincula. Segmental branches from adjacent digital arteries supply the vincula. Each flexor digitorum profundus (FDP) tendon has a short vincula attached to the middle phalanx and a long vincula attached to the proximal phalanx. The superficialis tendon (FDS) also has a short vincula and a long vincula which both arise at the level of the proximal phalanx. The blood supply from each vinculum enters the sheath on the dorsal surface [28].
Tendon Healing
The ability of tendons to hold tension for prolonged periods of time is the result of very low oxygen tension requirements. Consequently, tendons exhibit slow metabolic rates and slower healing [27]. Understanding the factors involved in tendon healing is necessary to optimize outcomes during and following repair.
Similar to wound healing, tendon healing has been divided into three distinct, but overlapping, phases: an inflammatory phase, a matrix production phase, and a remodeling and maturation phase. The inflammatory phase occurs within hours of injury, after initial hemostasis and clot formation. Neutrophils predominate early, followed closely by monocytes and macrophages at 24 h to phagocytose necrotic material. Cytokine release signals tenocyte activation for production of collagen III and early angiogenesis. Tendon strength during the inflammatory phase is defined by the strength of the suture itself [29]. The matrix production phase commences after a few days and lasts for approximately 4 weeks. Collagen III production is highest during this phase, however, also highly unorganized. Self-renewing tendon stem cells (TSCs) have recently been identified as prominent cells involved in initial collagen production [30]. The remodeling and maturation phase begins around week 4–6 with a decrease in tendon cellularity and transition of collagen type III to collagen type I. Tendon tensile strength is gained during this phase. Collagen fibers become properly aligned in parallel to lines of tension and important collagen cross-linking occurs. The repaired tendon is considered to be at full tensile strength around 12 weeks [31]. Tendon healing continues up to approximately 1-year post injury [27].
Mechanical loading and early motion play important roles in tendon homeostasis and decreased adhesion formation and are, therefore, key considerations following tendon repair. Tenocytes are mechanoresponsive in that they can modify the extracellular environment during mechanical loading through formation and degradation of matrix proteins [26]. Immobilization following tendon repair has been shown to significantly decrease the strength of repair within the first 3 weeks [32]. It is likely that immobilization results in an upregulation of inflammatory gene expression [33, 34]. Notably, tendon use has been shown to increase the number of activated fibroblasts that aid in tendon repair and remodeling [35]. Numerous studies have shown that early mobilization increases tendon tensile strength and reduces adhesion formation [36–39]. The proposed mechanism of this is that physiological forces promote gene expression of type 1 collagen formation during healing and that tension causes the collagen to be deposited and aligned in a parallel fashion [40]. Excessive tendon loading, however, can result in tendon injury, highlighting the importance of proper loading to improve outcomes following tendon repair surgery [41].
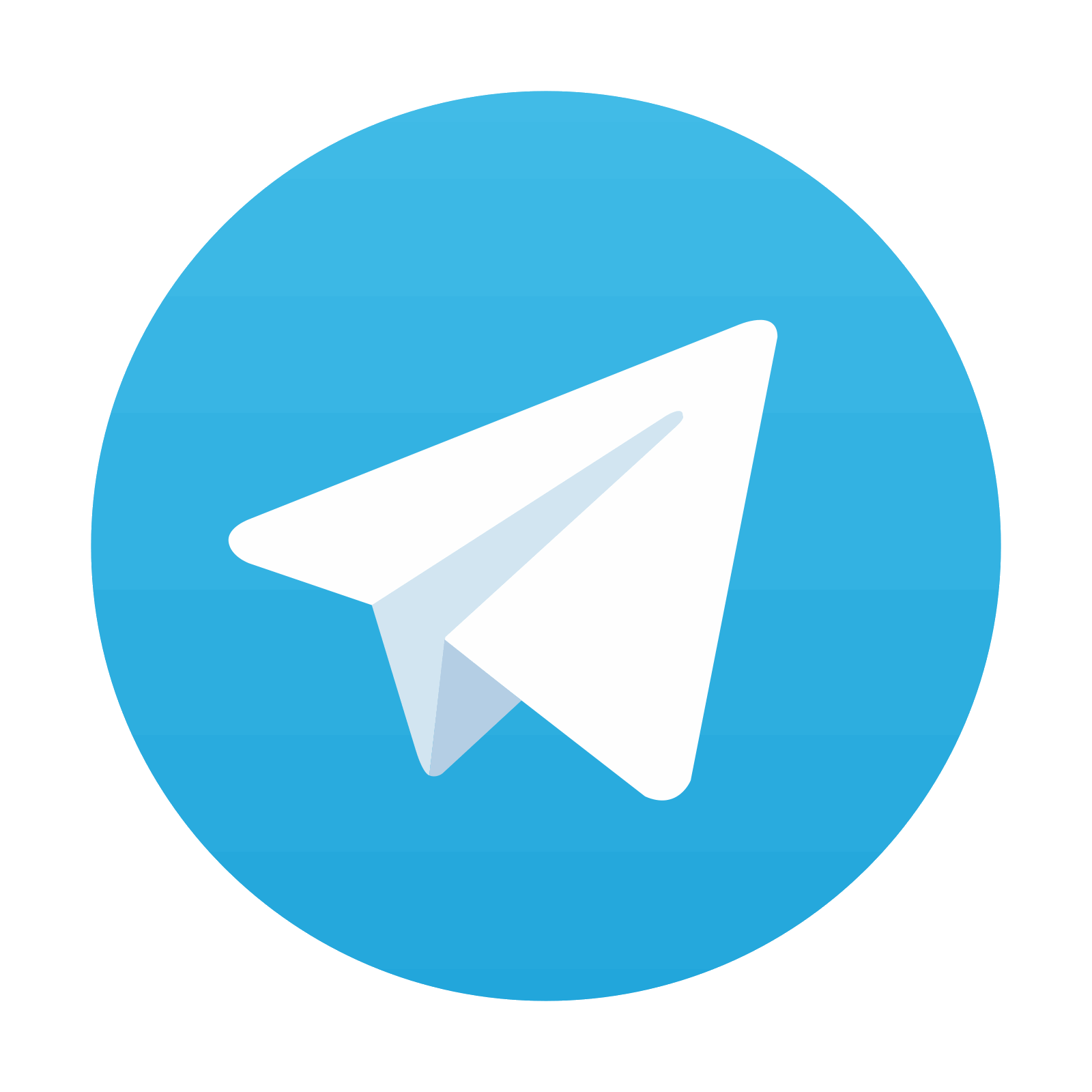
Stay updated, free articles. Join our Telegram channel
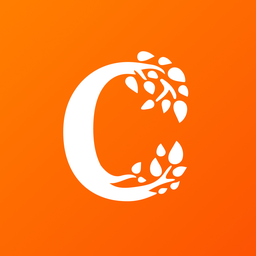
Full access? Get Clinical Tree
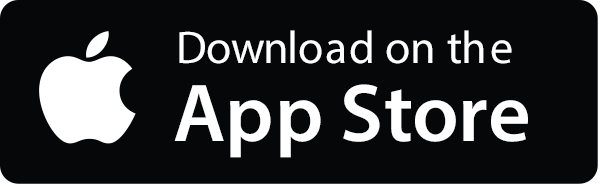
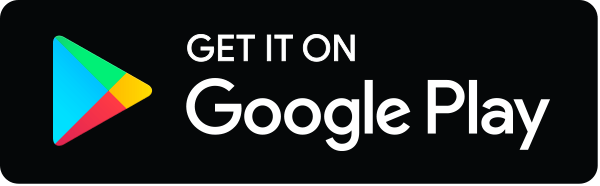