Abstract
Numerous surgical interventions have been developed and refined over the last few decades in an attempt to preserve the articular surface of the knee. Conservative options have more recently focused attention on injectable biologics in an effort to stimulate the body’s natural resources and create an intraarticular milieu suitable for healing. Reparative marrow-stimulation techniques can be used at the site of a chondral defect in an attempt to induce fibrocartilage repair tissue formation after penetration of the subchondral bone. Restorative cartilage procedures, by contrast, replace the native defect site with host or donor articular cartilage. This chapter focuses on the basic science of cartilage structure, discusses the aforementioned surgical and nonsurgical preservation techniques for the articular cartilage of the knee joint, and highlights expected future directions of study in the realm of surface chondral defect treatment.
Keywords
Autologous chondrocyte implantation, Cartilage, Knee, Orthobiologics, Osteochondral, Restoration
Introduction
Hyaline articular cartilage plays an integral role in the function of the knee joint. Isolated chondral lesions are incompletely understood, but once damaged, there is very little capacity for spontaneous healing due to intrinsically poor blood supply ( Fig. 9.1 ). Thus, the risk of patient pain, effusions, mechanical symptoms, decreased activity and quality of life, and the possibility of progression to diffuse osteoarthritis (OA) remain a concern. Between 30,000 and 100,000 chondral procedures are performed annually in the United States, and an annual incidence growth of 5% has been reported. The lesions are most commonly found in the medial compartment, followed by the patellofemoral compartment, and have been theorized to occur in approximately 12% of the population.

Numerous surgical interventions have been developed and refined over the last few decades in an attempt to preserve the articular surface of the knee. Conservative treatment options have more recently focused attention on injectable biologics in an effort to stimulate the body’s natural resources and create an intraarticular milieu suitable for healing. Reparative marrow-stimulation techniques–most notably microfracture–can be used at the site of a chondral defect in an attempt to induce fibrocartilage repair tissue formation after penetration of the subchondral bone. Restorative cartilage procedures (mosaicplasty, osteochondral allograft/autograft, particulated juvenile cartilage graft, autologous chondrocyte implantation [ACI]), by contrast, replace the native defect site with host or donor articular hyaline cartilage. These latter options have garnered more attention in the last decade as advanced efforts to provide pain relief, alter arthritic progression patterns, and hopefully delay or avoid arthroplasty.
Generally, varying specifications for use exists for each of the aforementioned procedures. However, no unified consensus exists on which cartilage repair or restoration technique exhibits the most successful long-term clinical outcomes. This chapter focuses on the basic science of cartilage structure, discusses the aforementioned surgical and nonsurgical preservation techniques for the articular cartilage of the knee joint, and highlights expected future directions of study in the topic of surface cartilage defect treatment.
Basics of Cartilage Structure
Cartilage is present in various parts of the human body and it is categorized into three different types: fibrocartilage, elastic cartilage, and hyaline cartilage. Each type has a unique function, structure, and composition. Hyaline cartilage, also known as articular cartilage, covers the articular surfaces between bones to provide a load-supporting, low-friction interface. This type of cartilage has low cell density and low proliferative activity and is avascular in nature, which makes innate regeneration nearly impossible.
Hyaline cartilage is primarily composed of water, chondrocytes, and an extracellular matrix (ECM). Chondrocytes are the cellular component of this type of cartilage and are highly differentiated cells with low proliferative activity. They are found in low abundance–only 1%–5% of cartilage by volume–but have high metabolic activity because they are responsible for maintaining homeostasis within the elaborate ECM. Mature chondrocytes lack cell–cell interactions and are instead surrounded by a pericellular matrix that extends radially from the cell surface. Chondrocyte function is affected by the surrounding environment including factors such as the compressive load within a joint, a phenomenon referred to as mechanotransduction. The ECM is composed of water and molecules including collagen, proteoglycans, and superficial zone protein. Water is the largest component of articular cartilage, responsible for 70%–80% by weight, and interacts with the extracellular components through its polar molecular structure to provide unique biomechanical properties.
There are more than 28 types of collagen identified within the human body. Type II collagen is the most prevalent type within hyaline cartilage and comprises approximately 50% of its dry weight. It is also a major component of the ECM. All types of collagen share a central core composed mostly of glycine, proline, and hydroxyproline causing the formation of a left-handed helix. These individual helices further assemble into right-handed triple helix microfibrils that form larger fibrils through end-to-end fusion and lateral bundling. These collagen fibrils are then arranged in different orientations in relation to the articular surface depending on their depth within the hyaline cartilage structure, and they provide stiffness to the tissue allowing it to bear weight.
The articular cartilage ECM also contains other molecules, the most prevalent of which are proteoglycans consisting of a protein core and many polysaccharides (primarily glycosaminoglycans [GAGs]) extending perpendicularly. GAGs are linear polysaccharides composed of repeating disaccharide units. The most common GAGs in hyaline cartilage are hyaluronan, dermatan sulfate, keratan sulfate, chondroitin 6-sulfate, and chondroitin 4-sulfate. Hyaluronan is unique in that it is the largest GAG, does not carry a negative charge, and is able to bind strongly with aggrecan–the main proteoglycan found in articular cartilage. The strong binding between hyaluronan and aggrecan results in the formation of large proteoglycan aggregates, and a fixed negative charge within the ECM causes a significant osmotic pressure in the cartilage’s interaction with synovial fluid. The end result is significant accumulation of fluid and swelling, known as the Donnan effect, that works with the collagen structure to produce the weight-bearing capability of articular cartilage.
Synovial fluid directly plays an important role in maintaining the articular cartilage. Synovial fluid is composed of protein-rich plasma ultrafiltrate and hyaluronan. As cartilage is avascular, the synovial fluid is responsible for providing nutrients through simple diffusion and compression–relaxation cycles during weight-bearing. It also contains a protein called “superficial zone protein”—or lubricin—which is also present on the surface of hyaline cartilage and contributes to the lubrication and ease of joint movement. Additionally, synovial fluid contributes to the load-bearing capacity by increasing its viscosity in response to pressure.
Articular cartilage is divided based on depth and composition into four structural zones: the superficial zone, the middle or transitional zone, the deep or radial zone, and the calcified zone. The outermost layer of cartilage is covered by the lamina splendens , which is a layer of proteins thought to be produced by the accumulation of proteins from synovial fluid that acts as a protective, low-friction layer for the cartilage. Immediately deep to that is the superficial layer of cartilage, which is densely packed with collagen fibers oriented parallel to the articulating surface and with a low concentration of proteoglycans. Chondrocytes in the superficial layer are flat in shape and also oriented parallel to the articulating surface. They produce proteins to lubricate the articular surface such as lubricin, which are not present in deeper zones. The middle zone is responsible for 40%–60% of cartilage thickness and has the highest concentration of proteoglycans. It has low cellular density, and its most prevalent ECM component is type II collagen arranged in arches. The chondrocytes here are round and produce a large amount of type II collagen and proteoglycans, specifically aggrecan. The deep zone has a lower cell density than the superficial or middle zones and contains type II collagen fibers oriented perpendicular to both the subchondral bone and articular surface. The chondrocytes in the deep zone appear elongated and are oriented parallel to the collagen fibers. Finally, the calcified zone contains hydroxyapatite and acts as a transitional zone between the cartilage and subchondral bone.
Injury to the articular surface can occur secondary to trauma of the joint causing disruption of the cartilage and formation of a focal chondral defect. The deeper cartilage layers, or possibly the subchondral bone, become exposed leading to pain, stiffness, and loss of function. If left untreated, focal chondral defects can progress to OA over time due to further degeneration of the surrounding cartilage. OA is caused by a combination of degenerative and abnormal remodeling processes within the cartilage in response to repetitive stress. Cartilage has low proliferative capacity making these processes nearly irreversible. Changes in the ECM begin in the superficial zone with the appearance of erosions, fissures, and fibrillation. The disruption of the collagen network results in a loss of proteoglycans that eventually inhibits its biomechanical function. The innate type II cartilage shows decreased fiber diameter while the type I cartilage concentration increases, representing the formation of fibrocartilage. Fragmentation continues until the subchondral bone becomes exposed, which allows direct force to be applied to the bone causing remodeling and thickening. Chondrocytes also undergo a series of changes during the development of OA including proliferation and pericellular matrix remodeling. Eventually, the chondrocytes die and release necrosis factors that induce apoptosis of surrounding chondrocytes. This leads to further degradation of the cartilage structure and eventual exposure of the subchondral bone.
Orthobiologic Injections
Hyaluronic Acid
Hyaluronic acid (HA) is naturally present throughout the human body but specifically is found within articular cartilage and synovial fluid. As OA progresses, the synovial fluid shifts toward lower-molecular-weight HA, leading to a decrease in its viscoelastic properties. Lower-molecular-weight HA is also strongly associated with higher levels of pain. Intraarticular HA injections have been used for many years as a treatment for OA directed at replenishing the concentration of HA and increasing the average molecular weight.
Intraarticular HA injections are most commonly believed to reduce symptoms of OA through mechanisms of chondroprotection. Within the joint, HA binds to cluster of differentiation 44 (CD44) and inhibits the expression of interleukin (IL)-1β, consequently inhibiting the synthesis of matrix metalloproteinases that have catabolic enzymatic activity toward collagen fibers causing the destruction of articular cartilage. The HA-CD44 binding pathway also augments chondroprotection through decreased apoptosis of chondrocytes, allowing preserved synthesis of the cartilage ECM and slowed degeneration. The current literature suggests that higher-molecular-weight HA is more effective at inducing these mechanisms of chondroprotection than lower-molecular-weight HA. Additionally, intraarticular HA injections have been shown to increase the synthesis and impair the degradation of aggrecan, thus slowing the progression of OA. Many studies have also suggested an antiinflammatory effect through decreased synthesis of IL-8, IL-6, prostaglandin-E 2 (PGE 2 ), and tumor necrosis factor-α (TNFα), in addition to the decrease in IL-1β. Some studies suggest a mechanical mechanism of action by increasing the viscosity of synovial fluid, which provides increased lubrication of the articular surface, and shock absorption. Few studies have reported that HA decreases the extent of subchondral bone changes in addition to functioning as an analgesic.
Intraarticular HA injections have shown variable outcomes in the current medical literature. Several studies and metaanalyses report statistically significant improvement in pain and function scores in patients with OA receiving HA injections while others suggest no difference between treatment and placebo. Also highly debated is whether the observed statistical difference is clinically relevant, as often times it has not exceeded the minimum clinically important difference (MCID). The efficacy of high-molecular-weight HA versus low-molecular-weight HA for treatment of OA has been discussed with some reports suggesting improved pain reduction with high-molecular-weight HA while others report no difference at all. While these studies have investigated the short-term benefit, recent literature suggests no difference in time to knee surgery or arthroplasty in patients receiving low-, medium-, or high-molecular-weight HA. Owing to the variable results in the medical literature, the current American Academy of Orthopaedic Surgeons (AAOS) guidelines state that a recommendation cannot be made for the use of intraarticular HA injections for OA.
Platelet-Rich Plasma
Platelet-rich plasma (PRP) is plasma containing supraphysiologic levels of platelets and platelet-derived growth factors used as a therapeutic modality for treatment of symptomatic cartilage defects and OA. PRP is produced from a patient’s venous blood that has been centrifuged to isolate the platelets, plasma, and growth factors ( Fig. 9.2 ). Platelets produce α granules, which contain many growth factors including transforming growth factor-β (TGF-β), fibroblast growth factor (FGF), and platelet-derived growth factor (PDGF). These growth factors have been found to be involved in tissue repair, and the goal is that PRP injections in theory could contribute to cartilage regeneration.

Recent in vitro and in vivo studies have shown that PRP functions by inducing cartilage regeneration and decreasing inflammation. Chondrocytes treated in vitro with PRP have shown increased proliferation and increased synthesis of type II collagen and GAGs. Additionally, in vitro studies have shown that PRP inhibits nuclear factor-κB (NF-κB), which is a transcription factor for the expression of proinflammatory and catabolic cytokines IL-1β and TNFα. In vivo, synovial fluid samples aspirated at 12 and 24 months after PRP injections trended toward decreased levels of IL-1β and TNFα, although the difference compared with treatment with HA was not statistically significant.
The existing literature varies in terms of PRP preparation technique, platelet concentration, white blood cell concentration, amount injected into the joint, and presence of an activating agent such as calcium chloride. The therapeutic range for platelet concentration is thought to be between two and six times higher than physiologic levels. A recent systematic review of six level I studies found significant improvement in clinical outcomes and Western Ontario and McMaster Universities Osteoarthritis Index (WOMAC) scores on OA patients treated with PRP when compared with HA at 3–12 months after injection. Very few studies have investigated outcomes past 1 year, but the available data suggest a decline in outcomes between 1 and 2 years after injection. The nuances of ideal PRP preparation to help maximize efficacy have begun to be elucidated in recent years, however. A systematic review of nine level I and level II studies that differentiated between leukocyte-rich and leukocyte-poor PRP found significant improvement in OA patients treated with leukocyte-poor PRP compared with HA or placebo but not with leukocyte-rich PRP. These data support the need for standardization of PRP preparations in order to maximize efficacy in all patients.
Bone Marrow–Derived Stem Cells
Mesenchymal stem cells (MSCs) have been of great interest for use in cartilage restoration and repair owing to their inherent regenerative potential. Bone marrow aspiration (BMA) has become one of the preferred techniques of acquiring MSCs, but stem cells only account for 0.001%–0.01% of nucleated cells in bone marrow. It is typically concentrated, usually through centrifugation, to produce bone marrow aspirate concentrate (BMAC) with higher concentrations of MSCs. Once concentrated, BMAC is then injected into the joint of interest either as an isolated treatment or augmentation to surgical treatment.
In addition to MSCs, bone marrow also contains high levels of growth factors and cytokines including vascular endothelial growth factor (VEGF), PDGF, TGF-β, and bone morphogenic protein 2 and 7 (BMP-2, BMP-7), which are known to have anabolic and antiinflammatory effects. Although PRP contains these same growth factors, BMAC has significantly higher concentrations. This mixture of growth factors has been identified to play a variety of roles in the cartilage regeneration capabilities of BMAC. VEGF and PDGF both promote angiogenesis, which increases the blood supply to the subchondral bone and normally avascular cartilage to promote regeneration. TGF-β and BMP both play a role in the chondrogenic differentiation of MSCs, which then synthesize type II collagen and GAGs. Collectively, the MSCs and accompanying molecules promote cartilage regeneration at the articular surface.
The results of intraarticular BMAC joint injections are promising both as an isolated treatment and as augmentation to procedures such as osteochondral collagen scaffolds. When compared with matrix induced chondrocyte implantation (MACI) for patellofemoral chondral lesions, significant improvement was seen in both groups, but MACI outcomes declined between years 1 and 2 whereas BMAC outcomes continued to improve. Additionally, chondral lesions treated with BMAC showed complete coverage in 80% of patients. When used in conjunction with collagen scaffolds to treat chondral defects, the repaired lesions showed better tissue similarity to surrounding hyaline cartilage by both magnetic resonance imaging (MRI) and histology compared with controls. As a whole, the existing literature suggests that the treatment of chondral lesions with MSCs in BMAC provides good outcomes as either an isolated or combined treatment.
Adipose-Derived Mesenchymal Stem Cells
Adipose tissue also contains MSCs termed adipose-derived mesenchymal stem cells (ASCs), which were first described in 2001. These cells have been found to have endodermal, mesodermal, and ectodermal proliferative potential, making them a great candidate to aid in cartilage restoration. ASCs are obtained via liposuction, and the adipose sample is then purified to isolate the stem cells. The stem cell concentration has typically been found to be significantly higher than that of BMAC.
Similar to both PRP and BMAC, ASCs have been shown in vitro and in animal studies to have antiinflammatory and chondroprotective characteristics. The exact mechanism has not been elucidated, but they appear to be activated by inflammation and in part modulate inflammation and cartilage remodeling through prostaglandin E2 (PGE 2 . The initial results of treating chondral defects with ASCs have been promising in relation to both clinical symptoms and lesion appearance. The first randomized control trial performed by Jo et al. found that intraarticular ASC injections in OA patients provide significant clinical improvement and cartilage regeneration observed by both MRI and second-look arthroscopy. There also appears to be a dose-dependent effect for ASCs that will be critical, as its preparation becomes standardized. Further investigation is needed to determine the long-term outcomes, but intraarticular ASCs provide a promising therapeutic avenue for symptomatic chondral lesions and OA.
Marrow Stimulation/Microfracture
Microfracture (MFx) is a common surgical procedure used in the treatment of focal chondral defects of the knee ( Fig. 9.3 ). The technique relies on marrow stimulation from the subchondral bone allowing the recruitment of MSCs for the formation of fibrocartilage repair. However, the outcomes of MFx surgery have been variable. Short-term clinical outcomes (<24 months) for MFx surgeries have been shown to have a high efficacy for small chondral lesions regardless of whether traumatic or degenerative etiology. A seminal systematic review including 3122 patients by Mithoefer et al. demonstrated that the average knee function scores remained above the preoperative level and that the short-term clinical improvement rate of MFx surgeries was 75%–100%. However, these same authors also demonstrated that the long-term outcomes of MFx showed reduced durability over time. After 24 months postoperatively, 47%–80% of MFx patients reported functional decline from their original improvements. Moreover, 67%–86% of subjects reported a decline in long-term improvement rate after 24 months. In another review by Steinwachs et al. , this decline in long-term clinical outcomes occurred even sooner (18 months postoperatively) in older patients and in patients with larger defects (>2.5 cm 2 ).

Long-term outcomes in high activity level patients, such as athletes, have also been questionable. A prospective study by Gobbi et al. followed athletes in order to measure their improvement after MFx. The participants demonstrated an improved Tegner activity scale at 2 years postoperatively, yet 80% of the athletes in the study progressively declined in sport activity at final follow-up. In two studies following National Basketball Association (NBA) patients who underwent MFx, a significant correlation was observed between MFx and decreased minutes per game, decreased player efficiency rating, or points per game. More importantly, 21% of the NBA players treated with MFx did not return to professional competition in the NBA. The predominant causal factors for poor long-term clinical outcome include inadequate clot stability and the concept that fibrocartilage is not the ideal replacement for defects in the articular cartilage, as it by comparison is soft and has a decreased ability to tolerate with shear stresses. Ultimately, this decreases the longevity and the outcomes seen with the MFx technique.
Recently, new innovations in augmentation strategies for MFx have been developed. A current advancement in MFx augmentation includes fixation of a collagen synthetic matrix over the drilled subchondral bone to act as an exogenous scaffold. The MSCs brought to the surface by MFx drilling interact with the collagen scaffold enhancing clot stability and MSC adhesion, organization, and differentiation into chondrocytes. The collagen-based scaffold’s intent is to promote and maintain the chondrocytic phenotype and type II collagen synthesis to ultimately fill the defect with regenerated natural hyaline-like cartilage. These MFx augmentation techniques seek to use potential autologous sources of cartilage regeneration in a fast, one-step, inexpensive procedure.
Most of the collagen scaffold augmentations of MFx provide equal or better short-term clinical outcomes when compared with MFx alone. Autologous matrix induced chondrogenesis (AMIC; ChondroGide), the arguably most well-studied collagen scaffold worldwide, uses a collagen type-III/I matrix bilayer matrix to serve as its natural scaffold. AMIC short-term outcomes at follow-up of 1 and 2 years have been demonstrated to be as effective as MFx. In a prospective randomized-controlled study by Anders et al., patients with a mean defect size of 3.4 cm 2 were randomized and treated either with MFx alone or an AMIC technique. Clinical outcomes (modified Cincinnati and International Cartilage Restoration Society [ICRS] score) were evaluated in 30 patients at 1 year and 27 patients at 2 years postoperatively. Clinical outcomes were significantly improved at 1 and 2 years postoperatively for all techniques used with no statistical difference between the techniques. However, AMIC has also exhibited promise in eliminating the two major weaknesses of MFx: long-term clinical outcomes and the ability to successfully treat larger size defects. The evidence for long-term clinical outcomes for AMIC is sparse, yet promising. A prospective randomized-controlled trial of 47 patients (mean defect size 3.6 ± 1.6 cm 2 ) treated either with MFx or AMIC demonstrated improved outcomes in all cohorts at 2 years postoperatively; however, a significant and progressive score degradation was observed in the MFx group. At that 2-year mark, only 11%–22% of all patients in the study described their knee function as abnormal, while at 5-year follow-up the percentage of MFx patients rose to 66%, whereas the percentage remained stable at 6%–7% for AMIC patients. Additionally, Schiavone et al. displayed a potential future for the use of AMIC in larger articular cartilage defects. The study examined a median defect size of 4.3 cm 2 (range, 2.9–8 cm 2 ) at median follow-up of 7 years. The results demonstrated a significant improvement from a mean international knee documentation committee (IKDCR) score of 31.7 (±8.9) points preoperatively to 80.6 (±5.3) and a significant improvement in Lysholm test when comparing preoperative score to final follow-up.
MFx and exogenous scaffolds can be further augmented by the use of PRP. Little is currently known about the addition of PRP to collagen graft augmented MFx procedures, but some evidence has shown that PRP can aid in recruitment of bone marrow MSCs from the underlying subchondral bone. BioCartilage (Arthrex Inc., Naples, FL) is a novel technique that combines a dehydrated allograft cartilage ECM scaffold and the addition of PRP. The ECM is made up of type II collagen, proteoglycans, and cartilaginous growth factors, which are components of native articular cartilage. Few peer-reviewed studies on BioCartilage outcomes are available, but in a study by Fortier et al. the authors reported that BioCartilage-treated knee lesions had significantly higher ICRS repair scores when compared with MFx alone at 2, 6, and 13 months postoperatively via repeat arthroscopy in equine models. Furthermore, when histology was examined, BioCartilage-repaired defects had significantly better formation of type II collagen than the control defects. The increase in type II collagen allows hyaline-like cartilage to regenerate within the defect, which is optimal for repair.
Chondrocyte Implantation Indications and Outcomes
While the aforementioned procedures result in fibrocartilage formation, which has biomechanical characteristics inferior to that of native cartilage, the following cartilage restoration procedures actually replace hyaline cartilage by cell-based implantation or osteochondral grafting, with or without the subchondral bone, using host or donor articular cartilage. These procedures include single-stage (mosaicplasty, osteochondral allograft/autograft, particulated juvenile cartilage graft) and two-stage (ACI) interventions. Not unexpectedly, regardless of technique, those patients who are younger, more active, with shorter preoperative symptom duration, fewer prior cartilage procedures, without concurrent ligamentous or meniscal deficiency, and with smaller isolated defects on the medial femoral condyle have the greatest expectations for superior outcomes. In the evaluation of these often complex patients, the orthopaedic surgeon must perform a thorough history and physical examination. It is necessary to identify the location and duration of symptoms, presence of knee swelling or instability, and a patient’s goals of care. Concomitant pathology of the meniscus, ligament(s), or mechanical axis must be addressed in a concurrent or staged fashion in order to provide a biomechanically sound environment for chondrocyte implantation surgery.
Osteochondral Autograft
Osteochondral autograft, also known as osteoarticular transfer system (OATS), includes whole-tissue transfer of cancellous autograft bone, normal subchondral bone tidemark, and mature hyaline articular cartilage, which immediately provides a new, functional chondral surface. This allows for a more rapid rehabilitation than the fibrocartilage maturation process of MFx or the cell-based maturation of ACI. The technology is beneficial in the treatment of full-thickness lesions. Either one single, large plug or multiple smaller plugs (known as mosaicplasty) of osteochondral tissue are transferred from non–weight-bearing areas (i.e., the periphery of the femoral condyles or superolateral/superomedial femoral trochlea) to the site of chondral loss. While osteochondral autografting can be technically difficult, its durability and successful outcomes particularly in high-demand patient populations makes it a popular option in the surgeon’s armamentarium. In general, clinical outcomes up to 17 years postoperatively have demonstrated good to excellent results in more than 90% of patients with defects between 1 and 5 cm 2 in size. However, morbidity including pain and discomfort at the donor/harvest site of the autograft is a concern.
Depending on defect location, size, ability to obtain perpendicular access, and surgeon experience, the lesions can be managed via all-arthroscopic or open techniques. The donor tissue is gathered by positioning the harvesting tool perpendicular to the cartilage surface, impacting to a depth of 10-mm, and removing the intact plug. The recipient site is prepared to accept the donor plug using a corresponding recipient core harvester, curettes, and/or motorized shavers to obtain stable vertical margins. The graft is gently inserted and impacted in a press-fit manner, so it is flush with the native surrounding cartilage. Harvest plugs should be limited to 3–4 cm 2 in size to avoid donor site morbidity and to allow the donor surface to reconstitute. After osteochondral autograft transplantation, the strongest MRI finding that correlates with clinical outcomes are defect fill and repair tissue structure, highlighting the importance of this reconstitution process. While osteochondral lesions between 1 and 8 cm 2 have been treatment by this technique, those lesions with <2 cm 2 are associated with superior outcomes.
Hangody and Fules reported their findings from 597 femoral condyles and 76 tibial plateaus treated by osteochondral autograft mosaicplasty. At up to 10 years postoperatively, 92% of patients undergoing femoral condyle treatment had good or excellent results, with 87% good/excellent findings for those with tibial plateau treatment. Solheim et al. found higher failure rates in patients who were women, over 40 years of age, and with defect size >3 cm 2 in their evaluation of 73 patients between 5 and 14 years postoperatively. Systematic review of nine studies with 607 patients by Lynch et al. demonstrated significant improvements, with return to sport as early as 6 months after surgery, and superior results for lesions <2 cm 2 . Pareek et al. systematically reviewed 10 studies with a total of 610 patients (mean defect size, 2.6 cm 2 ) with an average age of 27.0 years at the time of surgery. At a long-term mean 10.2 years’ follow-up, 72% of patients demonstrated successful outcomes, and the reoperation rate was 19%. IKDC and Lysholm scores improved significantly, but there was no improvement in Tegner score over the long-term despite a return-to-sport rate of 85%. The authors noted that increased age, greater numbers of previous surgical procedures, and increasing defect size correlated with risk of failure.
Osteochondral Allograft
Osteochondral allograft transplantation allows treatment of chondral lesions that are too large (>2 cm 2 ) to be effectively treated with OATS and can be performed in a single-stage unlike ACI ( Fig. 9.4 ). Plain radiographs are used for sizing purposes to find a matching donor. This allows for transfer of size-matched cartilage and subchondral bone into osteochondral lesions of the knee. It provides a good salvage option for failed prior cartilage restoration procedures as well. However, concerns with allograft use persist, including the risk for disease transmission, graft availability, technical difficulty, cost, and the long-term viability of cadaver chondrocytes and graft resorption. The highest level of chondrocyte viability is seen with fresh osteochondral allografts, with storage times ideally <24 days, and while frozen allografts demonstrate lower disease transmission rates, they additionally have inferior biological and biomechanical properties than their fresh allograft counterparts.
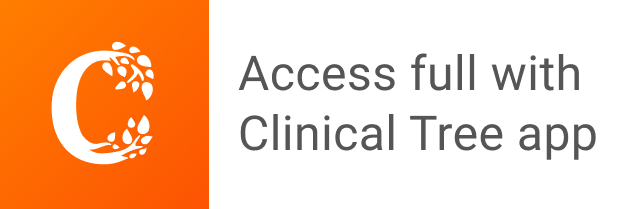