Fig. 2.1
The interaction of carbohydrates, triglycerides, and proteins, termed macronutrients, in the metabolic pathway. Note that all macronutrients have the capability to become Acetyl CoA, the common entryway to the oxidative energy cycle. Likewise, each macronutrient has the potential to become a different substrate in the metabolic process. Thus, metabolic precursors are highly interrelated. (Courtesy of Elizabeth A. Drum)
Muscle Fiber Types
Having reviewed macronutrient utilization in skeletal muscle cells in accordance with the energy pathways to produce ATP for sustained muscular action, we now transition to a discussion of muscle fiber types. Skeletal musculature is composed of slow- and fast-twitch (ST and FT, respectively) fibers or cells. ST are also known as type I or slow-oxidative (SO) cells and rely primarily on the aerobic energy system to produce ATP for muscle action. FT fibers, also known as type II fibers, are further categorized as FTa and FTx or type IIa and type IIx, respectively. FT fibers rely more on the ATP-PCr and anaerobic glycolytic energy pathways to sustain high intense action with a high rate of ATP production.
In general, type II muscle fibers are larger, transmit action potentials faster, have greater activity of the ATP splitting enzyme myosin ATPase, release and uptake calcium ions (Ca2+) more rapidly, and exhibit a high-rate crossbridge turnover (between actin and myosin or skeletal muscle myofibrils) than type I fibers, which exhibit all the aforementioned characteristics albeit at a protracted rate. Additionally, type II fibers tend to store more carbohydrate and are favored during high-intensity and/or power-strength exercises. Type I fibers, on the other hand, favor aerobic or low- to moderate-intensity exercise and rely on both carbohydrate and fat as fuel (note, both of these macronutrients are stored in type I fibers). Additionally, type I fibers have a greater concentration of mitochondria and capillaries versus type II fibers [5]. See Table 2.1 for a side by side comparison of the various fiber types.
Table 2.1
Skeletal muscle/fiber classification
System 1 | Type I | Type II | Type IIx |
System 2 | Slow twitch (ST) | Fast twitch a (FTa) | Fast twitch x (FTx) |
System 3 | Slow oxidative (SO) | Fast oxidative/glycolytic (FOG) | Fast glycolytic (FG) |
General attributes of fiber types | |||
Oxidative ability | Excellent | Good | Poor |
Glycolytic ability | Poor | Excellent | Excellent |
Contractile speed | Slow | Fast | Fast |
Fatigue resistant | Excellent | Good | Poor |
Unit strength | Poor | Excellent | Excellent |
Skeletal Muscle Force Production
We have seen that skeletal muscle physiology creates the opportunity for a plethora of muscle actions based on the combination of fiber types and energy pathways available . With the varied pathways to manufacture ATP, there is balance between low-energy requirements (e.g., rest or low-moderate-intensity exercise) and high-energy needs (e.g., high-intensity exercise). Notably, skeletal muscle can be selectively recruited to the muscular action needed based on fiber type and/or size of the fibers. Generally, as alluded above, type I fibers are smaller and therefore innervated by smaller alpha motor neurons, the primary neurons responsible for initiating muscular action. The size principle implies that during force development of progressively increasing magnitude, type I fibers are recruited first, followed by type IIa, and finally type IIx, the most powerful fiber set [6].
Therefore, the discussion about force production begins with acknowledging that the process of developing force in muscle is efficiently regulated, especially by increasing motor unit recruitment and increasing the frequency of motor unit discharge [5]. Recognize that a motor unit is, by definition, a single motor neuron and all the fibers it innervates—from a few to thousands [5]. The following section introduces the properties of skeletal muscle that allow it to exert force, namely the sliding filament theory (SFT) . Underlying the mechanics of force production are the omnipresent metabolic processes that maintain the ATP supply for sustainable muscle action and, therefore, force output. Said another way, you get work (W) output, which equals force (F) times distance (D) or W = F × D. This is a useful equation to remember when discussing muscular force production in that internal muscular force normally equals an external result, such as the movement of a weight stack or self-propulsion during walking or running .
A Few Muscle Action Definitions
A muscle fiber contraction or shortening of the muscle belly is a complex process that involves cellular and chemical interactions. The result is movement within the myofibrils in which thin (actin) and thick (myosin) filaments slide past and over one another. A muscular action may cause a shortening of a sarcomere , the smallest contractile unit of a muscle fiber, under tension (and ultimately a shortening of the muscle or contraction). This is termed as concentric muscle action. When the filaments slide in a direction causing a lengthening of the sarcomere under tension, an eccentric muscle action is created. When concentric and eccentric muscle actions are performed continuously, one after the other, such as during a full range of motion movement during a bench press exercise using free weights, this is termed isotonic exercise. Isometric muscle action, another relatable term, is created when thick and thin filaments attempt to slide in either direction, but a fixed or static length is held for a period of time. In other words, there is no lengthening or shortening of the sarcomere in a static action, even though energy is continuously being utilized to fuel the isometric event; maintaining your posture while sitting is a good example of static torso action. Another pertinent muscle movement to mention is isokinetic action in which muscle fibers exert a near constant, maximal force throughout a preset constant speed as seen in the use of an isokinetic Cybex machine in the rehabilitation setting.
Collectively, all the muscular actions mentioned above provide a brief overview of how specificity in resistance training methods can be incorporated with the principle of overload to create a thoughtful training or rehabilitation plan. Taken together, overload is the progressive increase in resistance on a particular muscle group over time in a systematic and specific fashion to elicit muscular force application and adaptation changes. Discussion of the role of resistance training in rehabilitation and performance training plans is covered in the later chapters of this book.
Sliding Filament Theory (SFT)
Working independently in 1954, H. E. Huxley [7] and A. F. Huxley [8] were involved in establishing the SFT . The theory explains how fixed-length thick (myosin) and thin (actin) filaments move in relation to each other, resulting in a change in muscle length and subsequently force production . In 1957, A. F. Huxley [9] further elaborated this model with a theory of crossbridge behavior which offered insights into how muscular force application is accomplished via internal, microscopic force application mechanisms. We have already established that metabolically force cannot be produced and sustained without macronutrient- or substrate-derived ATP. This primary energy structure is what interacts with muscle fiber myofilaments, actin and myosin, to allow the sliding filament model to “run” and crossbridge formation to unfold. Force happens when muscular action occurs (either concentric or eccentric). However, neurological input, discussed later in this chapter, is necessary to the initiation of muscle action via Ca2+ release into the sarcoplasm via specific structures. With that in mind, we turn to a few more characteristics of muscular force generation before returning to a more detailed discussion of the SFT.
Types of Muscular Action Relationships
Having introduced the SFT, where microscopic force application occurs, let us discuss a bit more about types of muscular interactions. Keep in mind that the term contraction can be misleading when discussing muscle properties and force-generating capabilities. Contraction of skeletal muscle seems to denote primarily a shortening of the fibers. However, in this chapter, we want to underscore that it indicates a shortening, lengthening, retention of the same length, or a combination of these depending on external loads and the desired activity. Therefore, a muscular contraction more accurately reflects a muscular action, than a contraction per se. Following are a few muscle fiber relationships that will help round out a review of muscular force production or the lack thereof.
Length–Tension Relationship
The length of a muscle fiber relative to its optimal length is critical in determining the amount of force or tension that can be developed. The optimal length can be defined as the length of the sarcomere , the smallest contractile unit of a fiber, that provides ideal overlap of thick and thin filaments [10]. Therefore, when optimal length is obtained in a sarcomere, normally around 90° in a joint, the greatest amount of force production is possible because of optimal actin–myosin interaction. Hence, when a muscle is shorter or longer than optimal length, there is impairment in maximal or optimal force production. For additional information concerning this type of relationship, especially in active muscle, the reader is directed to review Rassier et al.’s paper [11] titled Length dependence of active force production in skeletal muscle. Figure 2.2, below, illustrates various knee angles and the angular position that elicit the greatest muscular tension or strength.
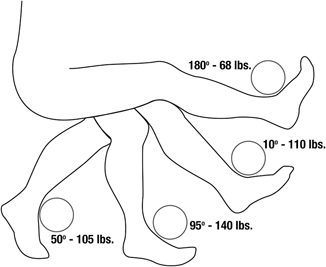
Fig. 2.2
Length–tension relationship of various joint angles, such as the knee joint, where at an angle of 95°, tension within fibers is highest or strength mobilization is greatest. Said another way, range of motion provides a basis for suboptimal and optimal crossbridge interaction. (Courtesy of Elizabeth A. Drum)
Force–Velocity Relationship
In accordance with the length–tension discussion, force–velocity variables also change the dynamics of skeletal muscle interaction. Simply stated, the force–velocity relationship indicates that as the speed of a muscular action increases, force production drops and vice versa [10]. This can be important during the rehabilitation of a patient or performance training of a client to insure that force development is optimized for whatever the outcome goal might be. For instance, to maximize force production in skeletal muscle , slower and controlled movement is probably warranted to optimize progressive overload manifested as greater force development in the musculature. On the other hand, if the goal is to improve speed or endurance, then it might be necessary to add a speed component to the performance of muscular actions along with understanding that force in the form of resistance needs to decline. In other words, the force–velocity relationship should guide training and rehabilitation to reflect that sport-specific or even every-day life activities are not slow and controlled but rather quick and sometimes unanticipated. Figure 2.3 showcases the force–velocity relationship.
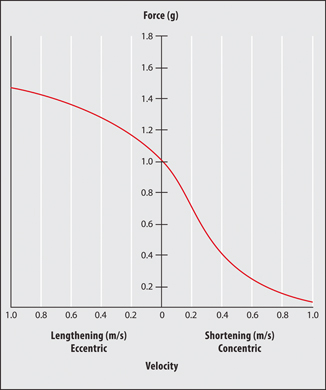
Fig. 2.3
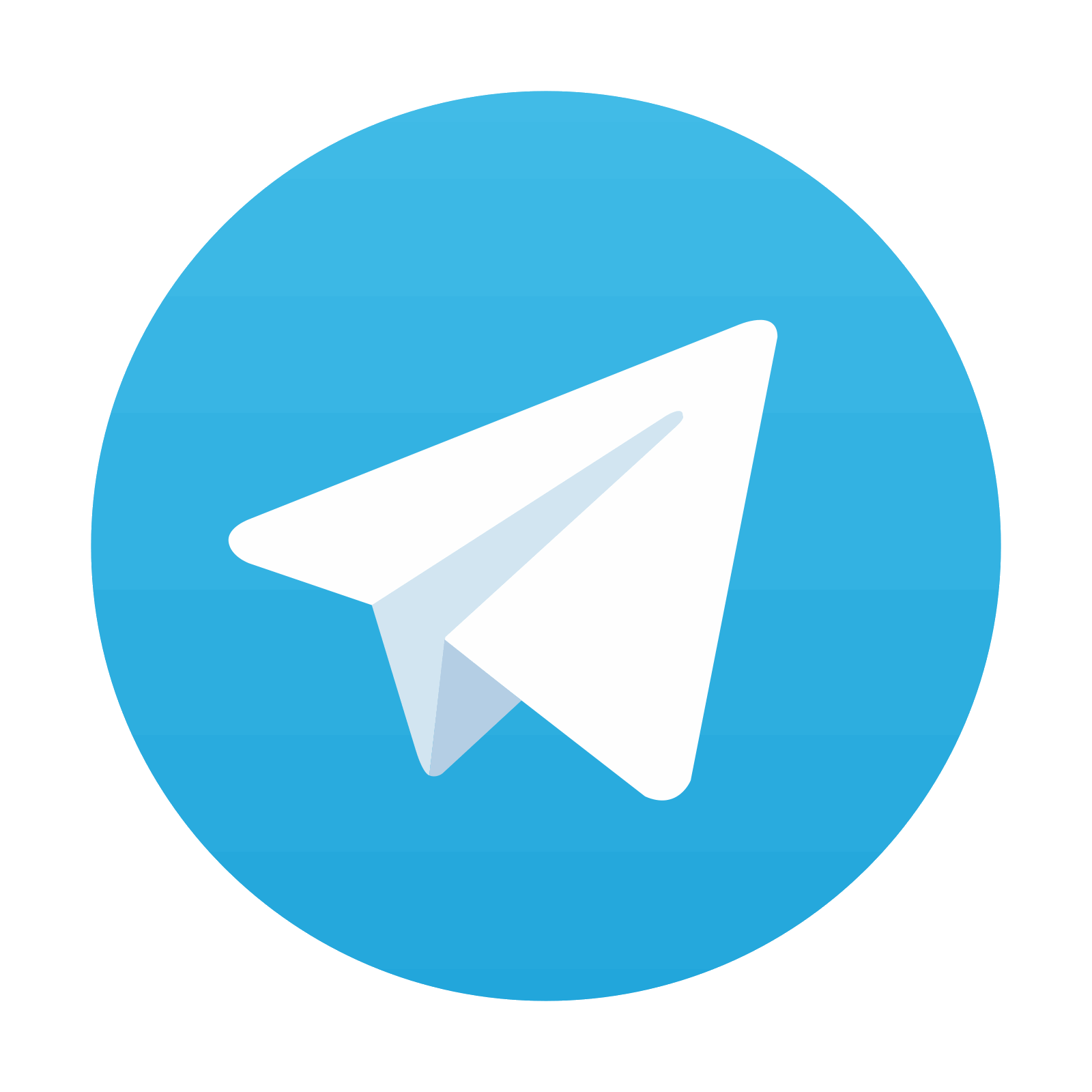
The force–velocity relationship of muscle action. During eccentric action, as the speed of fiber lengthening under tension increases, so does muscle tension. The opposite is true during concentric action or shortening of the fiber under tension whereby tension decreases as the speed of fiber shortening increases. (Courtesy of Elizabeth A. Drum)
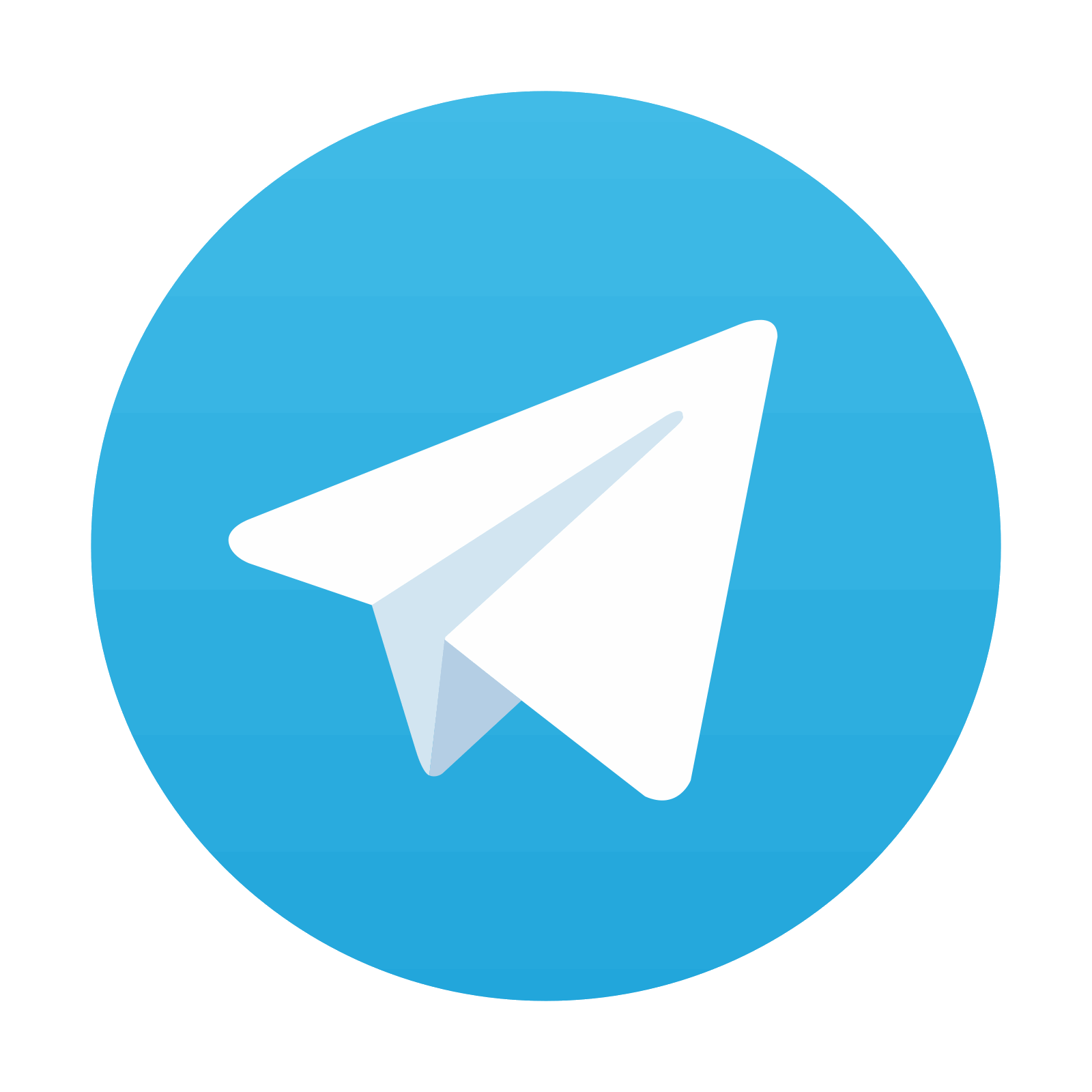
Stay updated, free articles. Join our Telegram channel
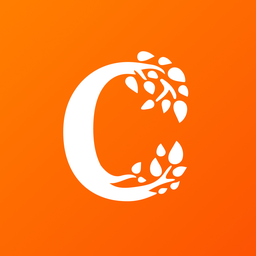
Full access? Get Clinical Tree
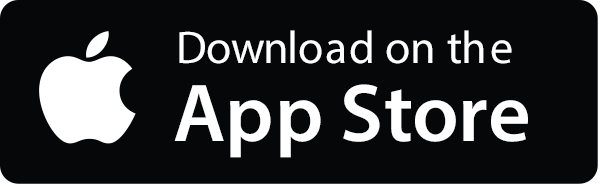
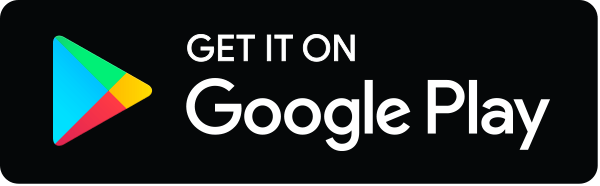
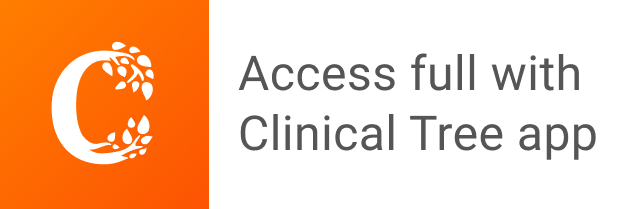