Fig. 43.1
Classification of spinal motion preservation technologies
Pedicle screw-based PDS systems have an advantage over total disk replacement in that they are based on techniques familiar to surgeons who have experience with posterior approach and traditional pedicular-based instrumented spinal fusions. The basic concept is to reduce the stiffness of the instrumentation to allow for load sharing between the instrumentation and the functional spine unit (FSU) at the instrumented levels [12]. Various implant designs have thus been developed to achieve this goal: reduction of diameter metallic rods, hinged pedicle screwheads that allow motion, damper components in the longitudinal elements, and more flexible rods made of non-metallic biomaterials.
Most PDS devices are approved for use as adjuncts to spinal fusion. However, inherent to their design, PDS systems are also utilized to address chronic low back pain by degenerative disk disease (DDD) and spinal stenosis. These devices may relieve symptoms of stenosis as well as discogenic pain by controlling motion, providing a certain degree of disk space distraction and indirect neurologic elements decompression, decreasing intervertebral disk stress, and unloading the facet joints. PDS devices may also reduce transmission of stress to the adjacent levels and potentially decrease the incidence of adjacent segment disease [11, 13–16].
The authors recently performed an extensive review of biomechanical and clinical investigations involving pedicle screw-based PDS devices currently available for use clinically [17–22]. The current chapter presents the most significant results from this review in both the biomechanical and clinical fields.
43.2 Biomechanical Concepts
To analyze the biomechanical effects of pedicle screw-based PDS devices, we have to distinguish two different indications: non-fusion (i.e., posterior dynamic stabilization) and fusion (i.e., dynamic fusion).
43.2.1 Dynamic Stabilization
The three basic biomechanical requirements for an ideal posterior dynamic stabilization device are [23]:
Unload the intervertebral disk evenly with a predictable load distribution
Control physiologic 3D motion (range of motion, ROM, and location of mean axis of rotation, AR)
Maintain/restore sagittal balance and anatomic alignment
43.2.1.1 Load Distribution
The intervertebral disk is composed of a homogeneous gel of collagen and proteoglycans and forms the main load-bearing structure in the FSU [24, 25]. Through experimental studies, the lumbar intervertebral disk demonstrated very high resistance to axial compression (ranging from 3,000 to 5,000 N) [24] with compressive loads on the nucleus redistributed evenly in a radial direction (Fig. 43.2a).


Fig. 43.2
Load distribution in the functional spine unit: in the normal (a), degenerative conditions (b), and following implantation of a posterior dynamic stabilization device (c)
Degenerative disk disease affects the ability of nucleus to bear compressive loads, and consequently more load is transferred to the peripheral regions of the disk and annulus (Fig. 43.2b) [4, 5, 26]. Profilometry in vivo studies by McNally [13] confirmed this abnormal load distribution in degenerative disks. In addition, disk degeneration results in the loss of disk height and increase of axial compressive loads to the posterior elements, potentially accelerating facet arthrosis.
Sengupta recently proposed that asymmetrical load distribution rather than abnormal motion was the main cause of mechanical low back pain [16]. This concept is supported by correlations between abnormal stress distribution across the disk space and painful disks on discography. The presence of high load zones has been described as the “stone in the shoe” theory by Mulholland [12]. Although current surgical treatment has focused on restricting intervertebral motion, abnormal transmission of load across the disk space is likely a significant source of low back pain. Similar process has been observed for hip and knee joints with asymmetrical load distribution resulting in accelerating arthrosis. Correcting osteotomy in the lower limbs has been therefore proposed to obtain more even load distribution.
Pedicle screw-based PDS devices have been designed as load-bearing devices to unload the degenerated disk and minimize load transfer to the posterior facets (Fig. 43.2c). By reducing the pressure on the degenerated disk and facets, pedicle screw-based PDS devices may reduce mechanical pain associated with these structures [15, 16, 27].
Load transmission through the functional spinal unit (FSU) following implantation of PDS systems has been investigated by finite element analysis and by measuring intradiscal pressure in cadaveric studies using flexible pressure transducers [28] (Table 43.1). Through these biomechanical studies, significant unloading of the intervertebral disk was observed, especially in extension and after distraction, whereas in flexion, intradiscal loads were similar to the loads of the intact spine.
Table 43.1
Load transmission at the instrumented level following implantation of PDS device and compared to the intact condition
Loading condition | Schmoelz et al. [29] experimental study | Zander et al. [30] FEA | Rohlmann et al. [31] FEA | ||
---|---|---|---|---|---|
Dynamic | Rigid | Dynamic | Dynamic | Rigid | |
Standing | NT | NT | ns | ↓ | ↓↓ |
Flexion | ns | ns | ns | ns | ns |
Extension | ↓↓ | ↓↓ | ns | ↓ | ↓↓ |
Lat bend | ↓ | ↓ | NT | NT | NT |
Axial rot | ↑ | ↓ | ns | ns | ns |
43.2.1.2 Kinematics
The intervertebral kinematics basically described the relative displacement of the upper vertebra with respect to the lower vertebra. The mean segmental flexion-extension range of motion (ROM) in the lumbar spine ranges from 10 to 18° depending on the vertebral level. In flexion-extension, the mean axis of rotation (AR) is located just below the surface of the upper endplate of the lower vertebra in the posterior one-third of the disk space (Fig. 43.3) [32]. The normal location of COR results from the natural tendency of the vertebra to move spontaneously in the path of least mechanical resistance.
In 1992 Panjabi defined “spinal instability” as an increase of the neutral zone on a load-displacement curve [33]. The neutral zone corresponds to the initial segment of the curve where there is low resistance offered by the FSU and subsequently great displacement occurs from minimal load application.
Although the relationship between low back pain and instability is not clearly defined yet, most authors accept that excessive non-physiologic motion may manifest as mechanical low back pain. According to the staged grading system of Kirkaldy-Willis, instability occurs in the early phase of disk degeneration, whereas the restabilization stage with reduction of motion and ankylosis of the FSU occurs in the late phase [34].
Results from experimental studies involving pedicle screw-based PDS devices [11, 35–39] support the biomechanical concept of “controlled motion without instability.” Scifert et al. has demonstrated in a calf model that PDS devices have the ability to provide significant stability with reduction of ROM compared to intact spines in flexion-extension and lateral bending [40].
By reducing excessive intervertebral motion, we can expect that implantation of such devices will result in relief of mechanical pain related to instability.
However, the biomechanical studies have demonstrated that pedicle screw-based PDS systems were more efficient to control ROM in flexion-extension and lateral bending than in axial rotation. This is important clinically as these devices may not be beneficial in cases of rotational instabilities, laterolisthesis, or scoliosis deformities. As examples, ROM following implantation of the DYNESYS® device ranged from 20 to 45 % of intact ROM in flexion, from 33 to 94 % in extension, from 26 to 40 % in lateral bending, and from 76 to 181 % in axial rotation [35, 41–44]. The mean ratio that was measured from experimental studies between DYNESYS® ROM and intact ROM is presented in Fig. 43.4.
Compared to soft stabilization devices, especially DYNESYS® implant which was the most investigated pedicle screw-based PDS device through experimental studies, few data are available concerning the effects on ROM following insertion of semirigid devices. Compared to soft PDS systems like DYNESYS®, these devices may probably result in a greater control in 3D motion, especially in axial rotation. That could justify their use in cases of deformity or instability. In case of degenerative spondylolisthesis, although Freudiger et al. found that DYNESYS® implant could control partially horizontal translation [41], semirigid devices may also be more suitable than soft PDS.
Restoration of normal segmental kinematics requires the return of ROM in physiologic range as well as physiologic quality of motion with respect to the location of the mean AR. The posterior placement of PDS systems is relatively far away from the physiologic axis of rotation. Subsequently, the posterior shift of the mean AR induced by most of the pedicle screw-based PDS results in a compression effect of the whole disk in flexion (anterior and posterior annulus) and a distraction effect, i.e., the unloading of the whole disk, in extension (Fig. 43.5b). This is in contrast to the normal kinematic behavior of the FSU (Fig. 43.5a).


Fig. 43.5
Consequences of posterior shift of the axis of rotation on intervertebral kinematics [17]. (a) Normal kinematics of the FSU in flexion-extension. (b) Kinematics of the FSU after implantation of pedicle screw-based PDS system
The non-physiologic kinematics following pedicle screw-based devices may result in overloading the intervertebral disk in some positions, especially when the spine is flexed (sitting position). Otherwise, some authors proposed the use of combination of PDS devices with total disk prosthesis anteriorly to deal with disk and facets degenerative changes [45]. According to us, there is a potential risk of conflict between the kinematic of the disk prosthesis and that of the PDS device. Although combination of the two types of motion preservation technology may be an attractive option, kinematic data suggest that such a combined construct may potentially result in overloading the disk prosthesis in flexion and complete unloading of the prosthesis in extension with the risk of dislocation.
More recently, using a nonlinear 3D finite element model of L4–L5, Jahng et al. [46] found that the center of rotation and stress distribution differed according to the design and materials used and also confirmed that the biomechanical effects induced by the implantation of dynamic stabilization systems produced non-physiological stress on the functional spine unit.
In 2014, using a 3D finite element model of the L4–L5 segment, Alapan et al. [47] confirmed that alterations in the location of the COR results in significant changes of load-sharing characteristics within the spine segment.
43.2.1.3 Alignment/Posture
It is now well accepted that restoration of sagittal balance with respect to anatomic spino-pelvic parameters is paramount in optimizing outcomes after lumbar fusion surgery [48–50]. Referring to classes of pelvis incidence may be particularly helpful to evaluate the amount of lordosis required in a spinal fusion procedure [51]. In general, restoration of adequate lordosis during fusion surgery is achieved by contouring the rod and then realigning the spine along the bended rod.
The use of flexible rods may therefore pose difficulties when trying to manipulate, correct, and maintain spinal alignment in the setting of spinal deformity, making this technology less optimal in these settings. Restoration of a large amount of lordosis may also be challenging.
In addition, most PDS systems provide a certain degree of posterior distraction to unload facet joints and posterior annulus and widen neural foramina potentially resulting in radicular and discogenic pain relief. However, posterior distraction may also lead to focal kyphosis and subsequently increased adjacent level stresses (Fig. 43.6).


Fig. 43.6
Potential risk of sagittal imbalance and hypolordotic construct with dynamic instrumentation due to the design of the rod
Legaye et al. reported a study on the unfavorable influence of pedicle screw-based PDS systems on the sagittal balance [52]. This author, who analyzed the impact of PDS implantation on sagittal alignment, noted that PDS devices were associated with loss of lumbar lordosis and pelvis backtilt, i.e., pelvis retroversion.
More recently, in 2011, Chen et al. [53] analyzed and compared the restoration of lordosis after implantation of hybrid versus purely dynamic instrumentation in the lumbar spine. Twenty-nine patients were included in the study. Local and global lordosis was measured using specific software. The authors concluded that hybrid construct could better preserve lordosis at the instrumented levels, therefore reducing the compensatory hyperextension of the adjacent spine above. Further study is needed to determine the efficacy of PDS devices in maintaining postoperative physiologic sagittal balance.
Otherwise, further clinical follow-up and correlation between pelvic morphometry (flat versus lordotic alignment) is necessary to better understand the consequences of such occurrences. The forces witnessed in the intervertebral disk depend partly on patient-specific spino-pelvic organization [50, 51] with predominant axial compressive forces noted in flat spines and predominantly shear forces in lordotic spines. Inherent to their design, pedicle screw-based devices are probably more suitable to control axial compression than shear forces, especially those with the presence of a spring/damper.
43.2.1.4 Implant Longevity and Adjacent Level
In contrast to traditional spinal fusion, dynamic devices must stay anchored to the bone in the setting of continued intervertebral motion. One of the complications that may therefore arise from instrumentation without fusion may be mechanical failure of the implant like loosening at the screw-bone interface or screw breakage [54]. Stoll et al. reported that rates of screw loosening, probably underestimated, were approximately 10 % in a series of 73 patients implanted with the DYNESYS® system (mean follow-up of 38 months) [55]. Benezech and Mitulescu recently reported the clinical and radiographical results of a series of 33 patients instrumented with the ISOLOCK® device without fusion [56]. After a mean follow-up of 45 months, they noted the presence of 5 mechanical complications (3 cases of screw breakage out of 148 screws implanted (2 %), 1 case of unscrewing of the screw nut, and 1 case of material loosening). However, there was no correlation between the presence of a mechanical complication and clinical results (the functional results were good or excellent in 76 % of patients with a return to previous work rate of 87 %).
Although mechanical failure of pedicle screw-based PDS devices may probably be unavoidable with time, such devices should have the advantage to delay the need of spinal fusion.
Otherwise, PDS offers a potential advantage over traditional rigid instrumentation in terms of adjacent segment load transmission [57]. However, to date, no clinical studies have reported their efficacy with regard to this objective. Additionally, some biomechanical studies did not find clear benefits of posterior dynamic stabilization in terms of minimizing adjacent level disease [29, 31, 44, 58]. As examples Schmoelz et al. [29] reported no difference in intradiscal pressure for both dynamic and rigid stabilization compared to intact spines, and Castellvi et al. [59] found only a 5.5 % reduction in maximal stresses provided by dynamic instrumentation versus rigid fixation at the adjacent level. These results suggest that the difference in the biomechanical effect between dynamic and rigid stabilization may not be as high as reported [58]. However, while the stress reduction may be small, it could be clinically significant because this effect is repeated over many loading cycles.
In 2012, Mageswaran et al. [60] reported results from a biomechanical experimental study involving seven human spine specimens and comparing three configurations: intact, one-level rigid instrumentation (L4–L5), and two-level hybrid construct (L3–L5) with dynamic stabilization at the level above (L3–L4). The idea was to evaluate the protective effect of the disk above (L3–L4) from excessive ROM and the creation of a transitional zone. The authors demonstrated that the biomechanical behavior of the dynamic instrumentation was very close to the rigid instrumentation. It reduced ROM at the above disk (L3–L4) but transformed one-level lumbar fusion into two-level lumbar fusion with augmentation of stresses and mobility at the supra-adjacent levels (L1–L2 and L2–L3).
Early clinical data suggests that results are as good as those reported using rigid instrumentation; however, further long-term follow-up studies comparing dynamic versus rigid stabilization are required to determine the efficacy of PDS on adjacent segment disease (Table 43.2).
Table 43.2
Biomechanics and pedicle screw-based PDS devices: key points
Capacity to unload the intervertebral disk in extension, lateral bending, and axial compression |
Kinematics: |
Control ROM in flexion-extension and lateral bending |
Posterior shift of the mean axis of rotation |
Only few data available concerning metallic semirigid devices |
DYNESYS® implant has been the most investigated device |
Risk of hypolordotic construct (inherent to their design) |
Reduction of stresses at adjacent segments |
Implant longevity as a limitation (high risk of screw loosening) |
43.2.2 Dynamic Fusion
43.2.2.1 Introduction
Surgery for low back pain due to degenerative disk disease and/or facet arthritis can be divided into three main options (Fig. 43.7):


Fig. 43.7
Main strategies for low back pain surgery including the concept of dynamic fusion
Limitation of motion (i.e., dynamic stabilization using motion-preserving devices)
Restoration of motion (i.e. involving disk/facets replacement implants)
Intervertebral fusion (considered as the gold standard)
Each option requires specific and adequate instrumentation to achieve the aim of the surgery.
In almost all cases, intervertebral fusion is currently performed using traditional rigid instrumentation. However, some authors advocated using a less rigid instrumentation to enhance intervertebral fusion success, thus introducing the concept of dynamic fusion [61–63].
Historically, most pedicle screw-based posterior dynamic systems (PDS) were initially designed to improve interbody fusion success in combination with an interbody bone graft [17–21]. In fact, most PDS devices are currently approved in the USA and Europe for use as adjuncts to spinal fusion and not as dynamic stabilization system. What is confusing is that these technologies are most often used as non-fusion devices (dynamic stabilization). Most papers and clinical reports on pedicle screw-based PDS concerned the concept of dynamic stabilization without fusion. Using the following keywords in a PubMed search, “lumbar dynamic instrumentation and fusion” and “Dynamic fusion and lumbar spine,” we found 47 papers on dynamic instrumentation, including 22 clinical reports, 14 biomechanical studies, and 5 reviews. All the papers involving pedicle screw-based PDS focused on the concept of dynamic stabilization but none on dynamic fusion.
43.2.2.2 Concept of Dynamic Instrumentation for Fusion
Dynamic instrumentation for fusion has been introduced in the 1990s to address the adverse effects of traditional spinal fusion observed with rigid instrumentation: pseudarthrosis, bone rarefaction, and mechanical failure.
Some authors suggested that eliminating mechanical loads on an interbody bone graft may result in negative bone remodeling, pseudarthroses, and osteoporosis [23, 64, 65]. This “stress-shielding” phenomenon at the disk space level may result from the excessive stiffness of traditional rigid instrumentation. Reducing the stiffness of the instrumentation, pedicle screw-based PDS allows for load sharing between the instrumentation and the functional spine unit (FSU) at the instrumented level(s). Using a finite element model of the lumbar spine, several authors demonstrated that posterior dynamic instrumentation, compared to rigid instrumentation, increases the amount of load transmission through the anterior column and the interbody bone graft, thus avoiding stress-shielding phenomenon. This may favor osteogenesis and enhance interbody fusion in accordance with Wolff’s law according to which the bone will adapt to the loads it is placed under, i.e., the structure and shape of bone permanently adapt to the loading conditions [66, 67]. Overload exposes to the risk of graft osteonecrosis, whereas underload may result in bone graft resorption. Thus, the basic concept of dynamic fusion is fewer loads through the instrumentation and more loads through the interbody bone graft without comprising stability, i.e., load sharing versus stress shielding.
In 1993, Lavaste and Perrin (unpublished data), using a finite element model of the lumbar spine, confirmed that dynamic posterior stabilization with ISOBAR TTL ™, compared to rigid instrumentation, increases the amount of load transmission through the anterior column (Fig. 43.8).


Fig. 43.8
Finite element analysis illustrating load-sharing phenomenon using posterior dynamic instrumentation (right) versus traditional rigid system (left) (From F Lavaste and G Perrin with permission, 1993, Laboratory of Biomechanics, ENSAM, Arts et Metiers Paristech, Paris, unpublished data)
Through a finite element analysis (FEA), Duffield et al. [64] compared the effects of three different longitudinal devices (4.8 and 6.3 mm rods and plate). They found that the axial load passing through the FSU was greater with 4.8 mm rod compared to 6.3 mm rod and/or plates (90 % versus 77 %, respectively). By using a canine model, Lim et al. [68] demonstrated that a less rigid stabilization device could reduce device-related osteopenia in the stabilized spinal segments and around the pedicle screws. In 1998, Templier et al. [65] using a 3D geometric FE model of the lumbar spine postulated that the TWINFLEX® semirigid device could offer a more favorable biomechanical environment for enhanced interbody fusion healing (Fig. 43.9). They evaluated the role of the longitudinal component in load transfer between the FSU and implant and noted that by reducing the stiffness of lumbar fixation, there was more homogeneous load transmission throughout the FSU without significantly reducing the rigidity of the instrumented spinal segment.


Fig. 43.9
In flexion, predominant load transfers through the system depend on instrumentation stiffness: a dynamic system results in anterior compression and posterior traction, while a rigid system results in axial pullout forces at the ends of the construct (Adapted from Templier et al. [65])
Finally, Goel et al. [61] developed a 3D finite element model to compare the load distribution of a hinged-dynamic posterior device versus a rigid construct and confirmed that the dynamic system enabled more load to be transferred through the anterior column as compared with traditional rigid instrumentation without comprising stability.
Theoretical biomechanical advantages of dynamic instrumentation for fusion are summarized in Table 43.3. These advantages may result in increase fusion rates, limitation of bone rarefaction, and reduction of mechanical complications with the ultimate objective to reduce reoperations rates.
Table 43.3
Theoretical advantages of dynamic instrumentation for fusion: key points
Load sharing between the instrumentation and interbody bone graft |
Avoid stress-shielding phenomenon in the anterior column |
Stresses reduction at bone-to-screw interface |
Less rigid fused segment |
Presently, most pedicle screw-based PDS devices are FDA approved as an adjunct for spinal fusion. Although controversies remain regarding the support of such a classification, pedicle screw-based PDS systems are classically divided into semirigid rod systems and tension-band posterior-based systems used as a non-fusion technology [4–6, 10–12, 14, 16]. Only semirigid PDS systems could logically serve for dynamic fusion since excessive flexibility provided by soft stabilization PDS devices may allow for excessive motion and excessive anterior loading of the interbody graft, resulting in endplate failure, subsidence, decreased fusion rates, and sagittal plane deformity (flat back). Consequently, a classification of pedicle screw-based PDS devices based on motion restriction is needed to separate soft from semirigid rod systems with which a fusion is generally intended.
43.2.2.3 Biomechanical and Clinical Experience with ISOBAR TTL™ Technology for Fusion
Implant Design
The basic concept of posterior dynamic systems is to reduce the stiffness of the instrumentation to allow for more physiologic load transmission at the instrumented levels. Various technologies have been introduced to allow for partially controlled three-dimensional motion or micromotion: reduction of diameter metallic rods, hinged pedicle screwheads that allow motion, damper components in the longitudinal elements, and more flexible rods made of non-metallic biomaterials [19–21].
In the literature, pedicle screw-based PDS devices are divided as follows: metallic rod systems (considered as semirigid stabilization), tension-band posterior-based systems (considered as soft stabilization), and hybrid devices.
ISOBAR TTL ™ consists of a metallic semirigid pedicle screw-based PDS made of titanium (minimum artifacts on MRI and CT) (Fig. 43.10) [21].


Fig. 43.10
ISOBAR TTL™, 1997, evolution of ISOLOCK® device 1993, Scient’x – Alphatec, France (Reprinted with permission)
It contains a damper element in its longitudinal element, a 5.5 mm titanium alloy rod. The damper, i.e., the dynamic component, allows reduced stiffness and limited amount of angular and axial micromotion (Figs. 43.11 and 43.12).



Fig. 43.11
Stacked washers within the dynamic component (From Scient’x – Alphatec, France, reprinted with permission)

Fig. 43.12
Range of motion provided by ISOBAR TTL™ implant (Reprinted with permission)
The damper provides ±2.25° angular ROM in flexion-extension and lateral bending, no limitation in axial rotation (unconstrained), and ±0.4 mm axial ROM (Fig. 43.12).
Concerning the surgical technique, this implant requires the same procedure as fusion performed with standard instrumentation using pedicle screws and rigid rod. Due to the familiarity of spine surgeons with pedicle screws placement, the learning curve for the implantation of the device is practically nonexistent.
In Vitro Testing
To support a classification of pedicle screw-based PDS systems, based on motion restriction, in vitro experimental investigations are needed. Concerning ISOBAR TTL ™ device, the experimental evaluation was performed by N’dri in the laboratory of biomechanics, Arts et Metiers Paristech, Paris, France (unpublished data).
Six human L2–S1 spinal specimens were tested intact, injured (laminectomy at L4–L5), and instrumented at L4–L5 using ISOBAR TTL ™ implant. Biomechanical tests were carried out using an optoelectronic system. Loads were applied to the upper vertebra (L2) with the lower vertebra (S1) fixed in a container. Pure moments were applied in flexion-extension, torsion, and lateral bending. Linear and angular displacements were measured using reflective markers rigidly fixed on L4 and L5 vertebrae (Fig. 43.13).


Fig. 43.13
Testing device
Results for instrumented spines in terms of range of motion, compared to intact spines, are presented in Fig. 43.14.


Fig. 43.14
In vitro evaluation of ISOBAR TTL™
Through this experimental investigation, the authors found that ROM following implantation of the posterior dynamic implant ranged from 20 to 50 %, depending on the loading condition. These results suggested that semirigid devices provide a greater control in 3D motion, especially in axial rotation, in comparison with results reported for soft stabilization devices.
Clinical Experience
To the best of our knowledge, one of the first introduced semirigid rod is the ISOBAR TTL™ implant (1997, evolution of ISOLOCK® device 1993) which has been now used in Europe for over 15 years and was approved FDA clearance for use as an adjunct to spinal fusion in 1999.
The first clinical implantation of the ISOLOCK™ device was performed by G Perrin in June 1993. In 1996 he published a report on the usefulness of intervertebral titanium cages for PLIF and dynamic posterior fixation. Patients were treated with semirigid ISOLOCK® plates for lumbar degenerative disk disease and spondylolisthesis. The fusion rate was more than 95 % without any mechanical failure of the instrumentation (Figs. 43.15 and 43.16).



Fig. 43.15
Case 1: interbody fusion obtained at L4–L5 using semirigid posterior dynamic stabilization (arrow) in combination with interbody bone graft (PEEK cages, oval shape)

Fig. 43.16
Case 2: L2–L3 severe stenosis treated by L2–L3 interbody fusion using semirigid posterior dynamic stabilization in combination with interbody graft (PEEK cages). Simple interlaminar decompression without diskectomy was performed at L3–L4
Unfortunately, there is no prospective study available comparing rigid versus dynamic instrumentation for fusion in the lumbar spine. Only case series have been reported in the literature [21, 63, 69]. The largest series has been reported by G Perrin (800 patients implanted with ISOBAR TTL ™); however, this author unfortunately mixed in his series patients with dynamic stabilization (no fusion), dynamic fusion, and hybrid constructs (rigid + dynamic) making the results difficult to assess. He retrospectively reported an overall fusion rate of 98 % with no mechanical complications.
There is no data available in the literature concerning the fusion period and/or the bone graft volume comparing dynamic versus rigid instrumentation.
In fact we consider dynamic instrumentation as an option to treat degenerative disk disease for given indications and given lumbar levels. The use of metallic rods with dampers may pose difficulties when trying to maintain spinal alignment or to restore a large amount of lordosis. Because of these sagittal balance considerations [48, 49, 51, 52], we estimate that dynamic instrumentation should be avoided at L5–S1 level. The authors feel that the best indications correspond to one or two levels to be fused between L2–L3 and L4–L5 (Fig. 43.17). To restore sufficient segmental lordosis with ISOBAR TTL™, it is essential to apply compression between the screwheads along the rod.


Fig. 43.17
A 55-year-old man presented with severe back and leg pain, not responding to conservative treatment. Imaging revealed a degenerative stenosis in L1–L2 and L2–L3 in relation with an L2–L3 disk hernia and inflammatory DDD at L1–L2. He was treated by decompression, followed by PLIF on two levels with cages and dynamic instrumentation. Surgical treatment provided a significant extent of immediate symptoms relief. One year after, a CT scan exam showed a solid fusion. Pain and disability levels decreased to 3/10 and 28/100, respectively
Otherwise, when using dynamic instrumentation for fusion, the authors’ preference is to place systematically the bone graft trough the intervertebral space (PEEK cages) rather than to realize an interlaminar and/or inter-transverse graft. Although some authors advocate the use of dynamic instrumentation in combination with posterolateral bone graft, we consider that dynamic instrumentation associated with interbody graft is more pertinent from a biomechanical point of view.
43.2.2.4 Conclusion
In comparison with the cervical spine, dynamic anterior cervical plates have been progressively introduced to provide a better graft loading with the ultimate objectives to accelerate spinal fusion and lead to a lower incidence of postoperative mechanical complications [70]. The use of dynamic instrumentation for fusion in the lumbar spine applies the same biomechanical concept, i.e., favoring load sharing versus stress shielding.
Further prospective studies are now needed to confirm the efficacy of PDS devices in enhancing spinal fusion and especially determine the advantages of dynamic instrumentation in terms of fusion period, fusion rates, and fusion quality.
43.3 Technologies and Classification of Pedicle Screw-Based Dynamic Stabilization Devices
The basic concept of PDS systems is to reduce the stiffness of the instrumentation to allow for more physiologic load transmission at the instrumented levels. However, the design of these devices varies greatly. In the literature, pedicle screw-based PDS devices are divided as follows: metallic rod systems (considered as semirigid stabilization), tension-band posterior-based systems (considered as soft stabilization), and hybrid devices [71].
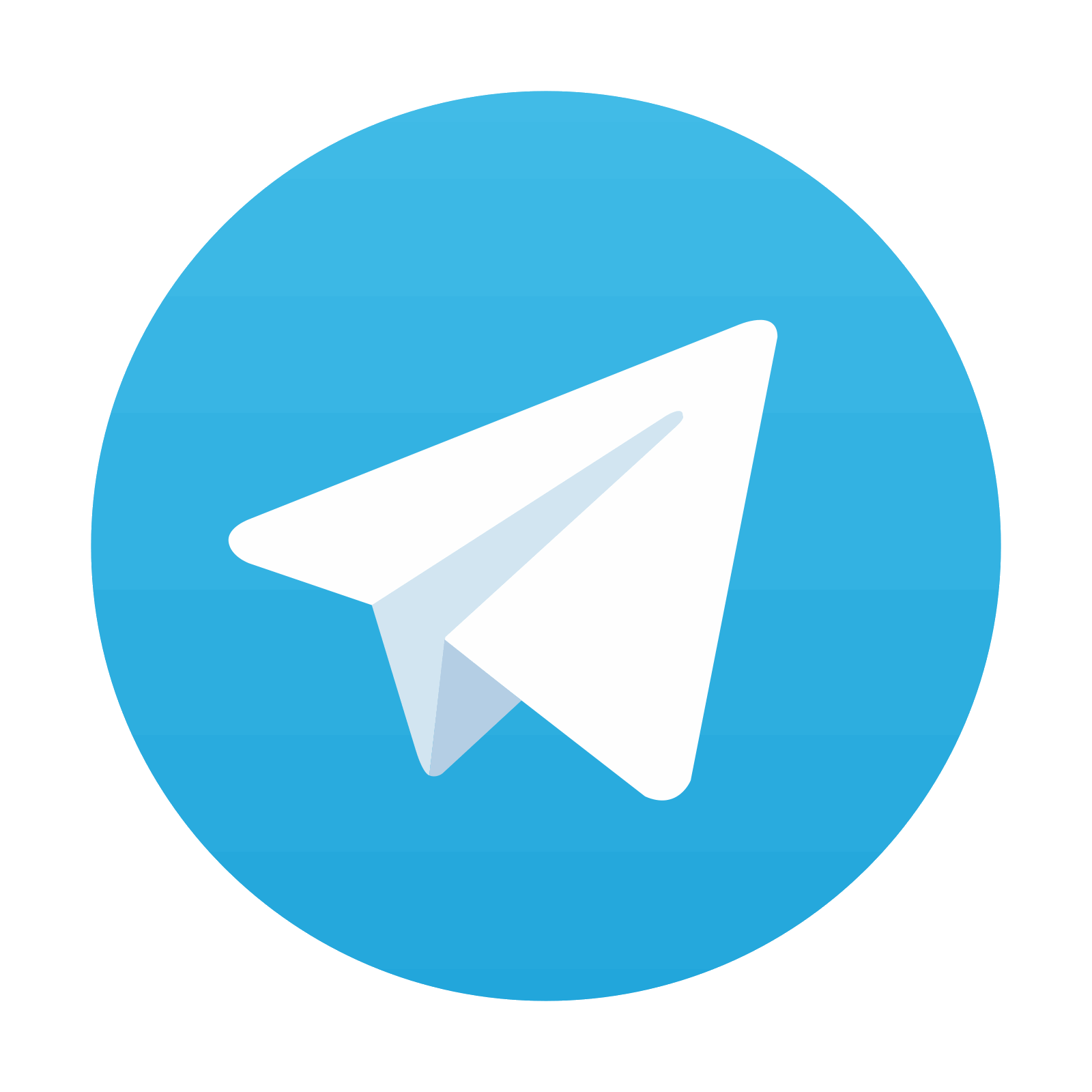
Stay updated, free articles. Join our Telegram channel
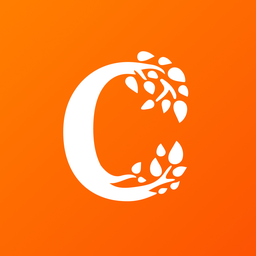
Full access? Get Clinical Tree
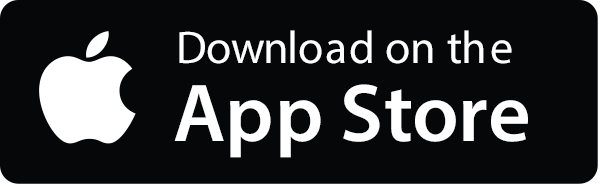
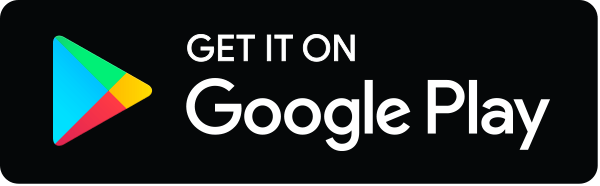