Fig. 1.1
(a) An illustration of two individual waves that are in phase. When summed together, the resulting wave is doubled. (b) An illustration of two individual waves that are 180° out of phase. When summed together, the resulting wave is completely canceled out
Mechanical fatigue is typically caused by the microdamage of myosin and actin bonds during eccentric muscular contractions [11]. As the amount of damaged myosin and actin increases, the ability to mechanically generate force is reduced. This will also cause a negative feedback loop into the neural component, thereby creating nonoptimal neural firing [10]. It is therefore clear that throwing is a high-velocity act that requires intricate neuromuscular control and timing to achieve optimal performance. In addition, the repetitive nature of throwing can easily lead to fatigue that will disrupt both the kinematics and kinetics. These components are the basis for understanding the pathophysiology of throwing injuries and will be discussed in more detail throughout this chapter.
Due to the complexity of the topic, we will incorporate both basic science and clinical principles to represent the full spectrum of understanding. The role of proper mechanics is very important and often difficult to master; therefore, both normal and abnormal throwing biomechanics will be covered in detail. In addition, the stress of throwing, even with proper kinematics, will cause structural adaptations to both the bone and soft tissues. These adaptations are often the key in both preventing and treating throwing athletes. Therefore, upper extremity structural adaptations will be discussed. Lastly, we will tie all of this information together to gain a more complex understanding of the clinical presentation of several common injuries that occur in throwing athletes.
Mechanics of the Overhead Motion: What Makes the Ball Go?
The overhead throwing motion is developed and regulated through a sequentially coordinated and task-specific kinetic chain of force development and a sequentially activated kinematic chain of body positions and motions [12]. The kinematics of the baseball throw have been well described and may be broken down into phases [13–15]. The most widely accepted descriptions of the phases of throwing include the wind-up, stride, arm cocking, arm acceleration, arm deceleration, and follow-through [15]. These descriptions portray how muscles can move the individual segments, demonstrate the temporal sequence of the motions, and describe the joint angles achieved. The shoulder has been shown to obtain between 160 and 185° of maximal external rotation and 14° of maximal horizontal adduction during the cocking phase, while humeral abduction reaches 90–95° at ball release during arm acceleration [14].
The kinetics have also been described. Moderate anterior shear (380 N) and compressive forces (660 N) occur during arm cocking with internal rotation and horizontal adduction torque reaching up to 90 and 110 Nm, respectively [14]. The forces and torques enable the high internal rotation velocity of approximately 7000° per second to occur during the arm acceleration phase. Consequentially, high posterior shear, inferior shear, and compressive forces occur (310–1090 N) as the body attempts to decelerate the arm [14]. These forces and motions are applied to all of the body segments to allow their summation, regulation, and transfer throughout the segments to result in the performance of the task of throwing. The muscle activation sequencing to produce these kinematics and kinetics demonstrates a proximal-to-distal activation to optimize efficiency [5, 16–19]. In the early phases of throwing (wind-up and stride phases ), scapular muscle activity (serratus anterior and upper trapezius) commences prior to larger global shoulder muscle activity (deltoid and pectoralis major) [17, 20]. As the throwing motion progresses from the stride phase to the arm cocking phase, the rotator cuff muscles, specifically the supraspinatus and infraspinatus, have a large amount of activity primarily to align the humeral head with the glenoid [20]. The high activity expands to the remaining rotator cuff muscles during the cocking phase in order to maintain concavity compression and to resist distraction [21, 22]. The cocking phase is also characterized by moderate to high concentric and eccentric activity in larger muscles such as the anterior deltoid, pectoralis major, latissimus dorsi, teres major, biceps brachii, and triceps brachii activity [22]. All of these muscles continue to work both concentrically and eccentrically throughout the remainder of the throwing phases in order to resist unnecessary translations, maintain proper positioning, and direct the ball to its target. The term “kinetic chain ” is used collectively to describe the mechanical linkages. Using these definitions and terminology allows a unifying concept to understand the overall mechanics.
An effective athletic kinetic chain is characterized by three components [23]: (1) optimized anatomy in all segments, (2) optimized physiology (muscle flexibility and strength and well-developed, efficient, task-specific, motor patterns for muscle activation), and (3) optimized mechanics (sequential generation of forces appropriately distributed across motions that result in the desired athletic function).
The kinetic chain has several functions: (1) It uses integrated programs of muscle activation to temporarily link multiple body segments into one functional segment (e.g., the back leg in cocking stance and push-off, the arm in long-axis rotation prior to ball release or ball impact) to decrease the degrees of freedom in the entire motion [13, 24, 25], (2) it provides a stable proximal base for distal arm mobility, (3) it maximizes force development in the large muscles of the core and transferring it to the hand [13, 26, 27], (4) it produces interactive moments at distal joints that develop more force and energy than the joint itself could develop and decrease the magnitude of the applied loads at the distal joint [5, 14–17, 28], and (5) it generates torques that decrease deceleration forces [14–16, 29, 30].
Multiple studies have clearly established the basic roles of the kinetic chain, both in baseball and tennis [5, 15, 17, 26, 28, 31–35]. Each body part has specific roles in the entire motion [13]. The feet are contact points with the ground and allow maximum ground reaction force for proximal stability and force generation. The legs and core are the mass for the stable base and the engine for the largest amount of force generation. The scapula must move in specific motions to provide a stable base for muscle activation and congruent ball and socket kinematics. During the initiation of throwing, the scapula is positioned in 40° of internal rotation in the plane of the scapula with slight anterior tilt [36]. As the phases progress, posterior tilt occurs until peaking at maximal humeral external rotation which then transitions to anterior tilt at ball release. Scapular external rotation occurs with maximal horizontal abduction which is likely why the highest serratus anterior activity is seen during the cocking phase [20, 36]. At ball release, the scapula begins in slight upward rotation but reaches a maximum of 40° upward rotation at humeral external rotation [36]. The high amount of lower trapezius activity coincides not as a prime mover of upward rotation but instead as a control for deceleration [22]. The shoulder is the funnel for force regulation and transmission and the fulcrum for stability during the rapid motion of the arm. The arm and hand is the rapidly moving delivery mechanism of the force to the ball or racquet.
To achieve its role in kinetic chain function, the shoulder must develop precise ball and socket kinematics to create maximum concavity compression [21] that optimizes functional stability throughout the entire range of rapid motion. Static restraints include the ligaments (at end ranges of motion) and the limited ball and socket anatomy of the humerus and glenoid. These static constraints must be limited to allow for the wide range of motions. Most of the constraints are dynamic, allowing wide ranges of motion but still conferring functional stability throughout the motions. Requirements for functional stability include optimum alignment of the humerus and glenoid within ±30° angulation [30], co-contraction and compression force couples of the rotator cuff and shoulder muscles [20, 37], a stable scapular base [38], adequate balanced rotational range of motion [39–41], and labral integrity to act as a washer, allowing “best fit” of the humerus into the glenoid [42].
Tasks performed in baseball and tennis occur as a result of the summation of speed principle which states that in order to maximize the speed at the distal end of a linked system, the movement should start with the proximal segments (the hips and core) and progress to the distal segments (shoulder, elbow, wrist) [16]. Each segment in this linked system can influence motions of its adjacent segments. For example, during a baseball pitch, stability of the back and stride legs allow rotation of the trunk which in turn allows for maximal throwing arm external rotation. The stable lower extremity serves as a platform for trunk and upper extremity motion where the amount of trunk rotation is proportionate to the amount of arm motion which can occur. Variations in motor control and physical fitness components such as strength, flexibility, or muscle endurance can affect the efficiency and effectiveness of all segments of the linked system [24, 25, 43].
Efficient mechanics can be improved by decreasing the possible degrees of freedom (DOF) throughout the entire motion [24, 25, 44, 45]. There are 244 possible DOF in the body from the foot to the hand [24]. Most models of maximum efficiency in body motions find that limiting DOF to about 6–8 maximizes the total force output and minimizes effort and load [45]. The DOF can be limited by coordinated muscle activation coupling, called integrative complexes, that constrain and couple positions and motions so that several segments move as one [44]. Examples include the back leg stance position in baseball cocking, where the body is stabilized over the planted leg [13], and the long axis rotation motion in baseball or tennis, where shoulder internal rotation, a minimally moving elbow, and forearm pronation allow the hand to rotate around the long axis from shoulder to wrist [34].
The limited number of independent DOF are called nodes and represent key positions and motions in the overhead tasks [13]. These key positions have been correlated with optimum force development and minimal applied loads and can be considered the most efficient methods of coordinating kinetic chain activation. There may be multiple individual variations in other parts of the kinetic chain, but these are the most basic and the ones required to be present in all motions. The baseball pitching motion can be evaluated by analyzing a set of eight progressive positions and motions (Table 1.1) [32]. These include trunk control over the back leg, hand in pronation “on top of the ball” in cocking, front leg directly toward home plate, control of lumbar lordosis in acceleration, hips facing home plate, arm cocking (scapular retraction/arm horizontal abduction/shoulder external rotation to maintain cocked arm in the scapular plane, “high” elbow above shoulder, and long axis rotation) coupled shoulder internal rotation/forearm pronation, at ball release [5, 13, 15, 17, 28, 31, 46]. The tennis serve motion can be evaluated by analyzing a set of eight “nodes” or positions and motions that are correlated with optimum biomechanics (Table 1.2) [13]. These include optimum foot placement, adequate knee flexion in cocking progressing to knee extension at ball impact, hip/trunk counter rotation away from the court in cocking, back hip tilt downward in cocking, hip/trunk rotation with a separation around 30°, coupled scapular retraction/arm rotation to achieve cocking in the scapular plane, back leg to front leg motion to create a “shoulder over shoulder” motion at ball impact, and long axis rotation into ball impact and follow-through [13, 23, 48]. These nodes can be evaluated by visual observation or by video recording and analysis. Tennis-specific pathomechanics with detailed descriptions of the deleterious motions are listed in Table 1.2.
Table 1.1
Baseball nodes and possible consequences
Node | Normal mechanics | Pathomechanics | Result | To be evaluated | |
---|---|---|---|---|---|
1 | Foot position | Directly toward home plate | Open or closed | Increased load on the trunk or shoulder | Hip and/or trunk flexibility and strength |
2 | Knee motion | Stand tall | Increased knee flexion | Decreased force to arm | Hip and knee strength |
3 | Hip motion | Facing home plate | Rotation away from home plate | Increased load on shoulder and elbow | Hip and trunk strength |
4 | Trunk motion | Controlled lordosis | Hyperlordosis and back extension | Increased load on abdominals and “slow arm” | Hip and trunk strength |
5 | Scapular position | Retraction | Scapular dyskinesis | Increased internal and external impingement with increased load on rotator cuff muscles | Scapular strength and mobility |
6 | Shoulder/scapular motion | Scapulohumeral rhythm with arm motion (scapular retraction/humeral horizontal abduction/humeral external rotation) | Hyperangulation of the humerus in relation to the glenoid | Increased load on the anterior shoulder with potential internal impingement | Scapular and shoulder flexibility and strength |
7 | Elbow position | High elbow (above 90° abduction) | Dropped elbow (below 90° abduction) | Increased valgus load on elbow | Scapular position and strength, trunk and hip flexibility and strength |
8 | Hand position | On top of the ball | Under or on side of the ball | Increased valgus load on the elbow | Shoulder and elbow position |
Table 1.2
Tennis nodes and possible consequences
Node | Normal mechanics | Pathomechanics | Result | To be evaluated | |
---|---|---|---|---|---|
1 | Foot position | In line, foot back | Foot forward | Increased load on trunk or shoulder | Hip and/or trunk flexibility and strength |
2 | Knee motion | Knee flexion greater than 15° | Decreased knee flexion less than 15° | Increased load on the anterior shoulder and medial elbow | Hip and knee strength |
3 | Hip motion | Counterrotation with posterior hip tilt | No hip rotation or tilt | Increased load on shoulder and trunk; inability to push through increasing load on abdominals | Hip and trunk flexion flexibility and strength |
4 | Trunk motion | Controlled lordosis; X-angle ~30° | Hyperlordosis and back extension; X-angle <30° (hypo), X-angle >30° (hyper) | Increased load on abdominals and “slow arm”; Increased load on the anterior shoulder | Hip, trunk, and shoulder flexibility |
5 | Scapular position | Retraction | Scapular dyskinesis | Increased internal and external impingement with increased load on rotator cuff muscles | Scapular strength and mobility |
6 | Shoulder/scapular motion | Scapulohumeral rhythm with arm motion (scapular retraction/humeral horizontal abduction/humeral external rotation) | Hyperangulation of the humerus in relation to the glenoid | Increase load on the anterior shoulder with potential internal impingement | Scapular and shoulder strength and flexibility |
7 | Shoulder over shoulder | Back shoulder moving up and through the ball at impact and then down into follow-through | Back shoulder staying level | Increased load on abdominals | Front hip strength and flexibility, back hip weakness |
8 | Long-axis rotation | Shoulder internal rotation/forearm pronation | Decreased shoulder internal rotation | Increased load on medial elbow | Glenohumeral rotation |
Adequate performance of the kinetic chain requires optimum anatomy and physiology. Optimum anatomy must be present in all of the joints in the kinetic chain. Joint injury (such as sprained ankles, unresolved knee injury or stiffness, hip tightness, or back injury) can have deleterious effects for core stability, force production, interactive moment production, and arm position [23, 43]. Optimum physiology requires adequate muscle strength, flexibility, and endurance throughout the kinetic chain. It also requires proper muscle activation patterns for core stability, force development, integrative complexes, joint stabilization, and segment deceleration [23]. The optimized anatomy can then be acted upon by the optimized physiology to create task-specific mechanics to achieve the kinematics and kinetics that produce the desired result of optimal performance in throwing or hitting the ball and create the lowest possible risk of injury.
Abnormal Biomechanics Caused by Structural and Neuromuscular Adaptations
Due to the large repetitive stress of throwing that was described previously, several tissues go through structural and neuromuscular adaptations. These adaptations are different for each tissue type, location, and function. Ultimately, these adaptations cause abnormal pitching biomechanics, which will increase the stress on tissues. This will cause a downward spiral effect, which leads to further tissue adaptations and additional alterations in pitching biomechanics. The combination and continual progression will ultimately lead to shoulder or elbow injuries, which commonly require surgical intervention. In this section, we will cover each of the adaptations that occur due to throwing and the effect they have on pitching biomechanics.
Range of Motion
The most common adaptation that is seen clinically in throwers is a shift in the arc of shoulder motion bilaterally. Throwers often present with a decrease in glenohumeral internal rotation (IR) and a concurrent increase in glenohumeral external rotation (ER) on the throwing arm compared to the nonthrowing arm [49–58] (Fig. 1.2). Wilk et al. [41] has developed the total motion concept which adds IR and ER together to calculate the total arc range on each side. Wilk et al. [41] states that if the total motion is equal bilaterally regardless of the shift in motion pattern, then the clinician should not be concerned. When total motion is equal bilaterally, it has been suggested that the shift in the arc of motion is only caused by a bony adaptation called humeral retroversion. However, if there is a loss of total motion on the throwing side, there is usually a soft tissue tightness present which may be reversible with treatment. Recently, this has been supported demonstrating that baseball players with a loss of total motion of 5° or more had a higher rate of shoulder injury [59, 60]. In addition to the total motion concept, there has been much research investigating glenohumeral internal rotation deficits (GIRD) . This is a term that has been developed to describe the loss of IR on the throwing arm [52]. There are several hypotheses for the cause of GIRD; however, evidence is still lacking to fully understand the specific tissue adaptations. The three main hypotheses are humeral retroversion, posterior rotator cuff tightness, and posterior capsule tightness/thickness. Each of these tissue adaptations will be discussed in detail in upcoming sections. Regardless of the source of GIRD, it was demonstrated that baseball players with a GIRD of 20° or more were two times more likely to be injured [59].
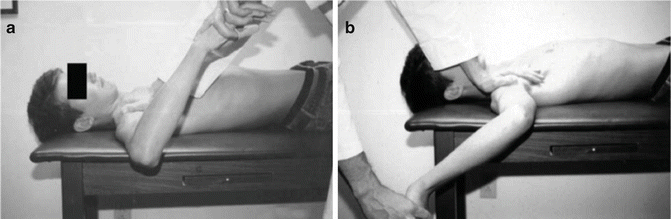
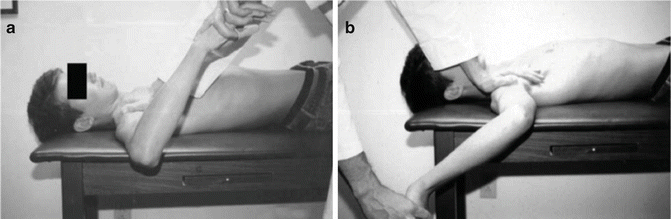
Fig. 1.2
(a) Internal rotation is measured with the patient’s shoulder in 90° abduction and the elbow in 90° flexion while the examiner stabilizes the scapula. The end point of internal rotation is taken as the point at which the scapula begins to rotate posteriorly. (b) External rotation is also measured while stabilizing the scapula. Note that the neutral position (0°) is that in which the forearm is perpendicular to the patient’s body (12 o’clock position in the supine patient) (Reprinted from Burkhart SS, Morgan CD, Kibler WB. The disabled throwing shoulder: spectrum of pathology Part I: pathoanatomy and biomechanics. Arthroscopy. 2003 Apr;19(4):404–20, with permission from Elsevier)
Bone
The bone is a tissue that is known to have adaptable properties to mechanical load [61]. As such, throwing causes several bony adaptations that are important in understanding the throwing athlete. First, humeral retroversion is described as the bony rotation between the proximal and distal ends of the humerus [62] (Fig. 1.3). Traditionally, it is measured with CT or MRI; however, ultrasound has been demonstrated to be as accurate and have much more accessibility [63, 64]. To understand humeral retroversion in throwers, we first need to discuss the developmental process in normal individuals. At birth, the humerus is in an excessively retroverted position [65]. Clinically this equates to increased glenohumeral ER and decreased glenohumeral IR [66]. Throughout normal development, the humerus undergoes a rotation process that decreases the amount of humeral retroversion. It has been shown that 80 % of the normal developmental rotation process is completed by 8 years old and the remaining 20 % extends to 18 years old [65]. Throwing prior to the completion of this normal developmental rotation process seems to diminish or halt the process, thereby creating side to side differences in humeral retroversion. Most throwers will have more humeral retroversion on the throwing side compared to the nonthrowing side. The association between humeral retroversion and injury is still being heavily researched. Initially, it was suggested to be a positive adaptation. Early researchers reasoned that increase retroversion would afford ER without stretching the anterior capsule and would theoretically inhibit tuberosity/glenoid contact in ER (internal impingement) [47]. However, some recent research suggests that baseball players with greater humeral retroversion have a history of elbow injury [67]. Others have found that baseball players with greater humeral retroversion have a thicker posterior capsule of the shoulder [68], which has been implicated in causing shoulder and elbow injuries [52]. Currently, the evidence suggesting that humeral retroversion is either helpful or deleterious to long-term performance is inconclusive.
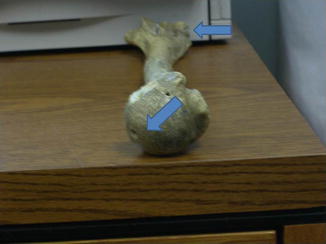
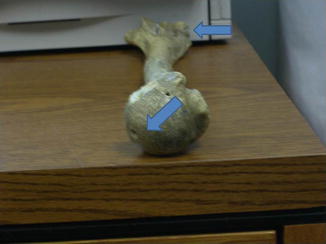
Fig. 1.3
Humeral retroversion (HRT). HRT can be measured as the angle formed by a line drawn through the center of the longitudinal axis of the humeral head and neck meeting a line drawn along the transverse axis of the condyles, when looking proximal to distal along the humerus (Reprinted from Kinsella SD, Thomas SJ, Huffman GR, Kelly JD 4th. The thrower’s shoulder. Orthop Clin North Am. 2014 Jul;45(3):387–401, with permission from Elsevier)
Next, bone mineral density has been examined bilaterally in baseball players. The results of these studies suggest that the proximal and mid shaft of the humerus on the throwing arm has increased bone mineral density [69, 70]. This finding would be hypothesized based on Wolf’s law [61] and the rotational stress of throwing. These results suggest that throughout development, the humerus will adapt in a manner equal to the mechanical loads that are placed on it. This information is important in adolescent throwers due to the open epiphyseal plate at the proximal humerus and the increased propensity for little leaguer’s shoulder. Adolescent throwers need to progress in throwing at a much slower pace and also limit the amount of pitches per game and season to allow the bone to adapt at a healthy rate.
The last bony adaptation that can occur in throwers is morphological changes to the bicipital groove. The bicipital groove can develop stenosis from bone ingrowth or spurs [71] (Fig. 1.4). The increased growth of bone within the groove will cause mechanical irritation to the synovial sheath of the biceps tendon and over time cause significant injury. Although bicipital grove stenosis hasn’t been documented much in the literature, it likely occurs more frequently in throwing athletes than has previous been suspected.
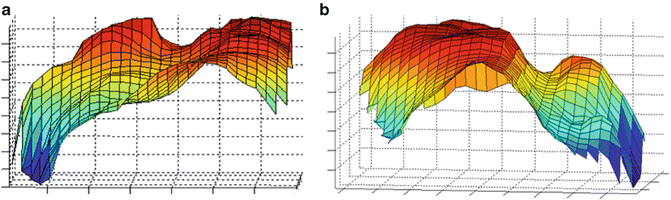
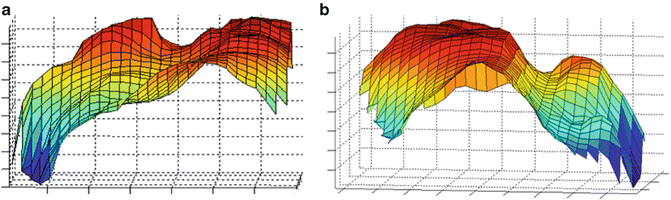
Fig. 1.4
A three-dimensional reconstruction of a bicipital groove viewed from distal to proximal through the bicipital groove. (a) This demonstrates the development of bicipital groove stenosis. (b) This demonstrates a normal bicipital groove area
Soft Tissue
There are many different types of soft tissues in the shoulder that can adapt due to the stress of throwing. Each tissue is different in terms of its composition, structure, and function. In this section, we will discuss all of the different types of soft tissue that are important when treating throwing athletes.
The first category is the joint capsule . The capsule is composed of an inner and outer layer. The inner layer is known as the synovial layer and is responsible for secreting synovial fluid to maintain joint health. The outer layer is composed of dense irregular connective tissue [72]. This layer provides the strength and stabilizing component to the capsule. Throwers can develop adaptations in both the anterior and posterior locations of the joint capsule [68, 73]. During the late cocking and acceleration phase of throwing, large anterior forces occur [14]. Due to these repetitively large forces, it is often thought that the connective tissue of the capsule plastically deforms and is left in a lengthened position. Structurally, the anterior capsule will be unable to center the humeral head on the glenoid at the end ranges of motion. This will allow increased joint translations in the anterior direction, which has been thought to cause secondary impingement or labral injury [74]. During throwing, the athlete will have excessive ER and pain during the late cocking phase of the throw. However, a detailed examination is necessary to discern whether the ER seen during throwing is caused by a combination of glenohumeral, scapular, thoracic, and lumbar motion. It is important to note that anterior capsular laxity does not occur to every throwing athlete. It is often used as a generic diagnosis due to the player having excessive ER and shoulder pain. For example, a recent study by Borsa et al. [75] demonstrated that healthy college throwers did not have side to side differences in anterior translation. This suggests that when this increased anterior humeral translation does occur, it is likely pathologic and may require surgical intervention.
Next, the posterior capsule also undergoes structural adaptations; however, these adaptations are much different than the anterior capsule. When examining pitching kinetics, research has shown that during the deceleration phase, the distraction force is on average 1.5 times body weight [14]. Typically, during the deceleration and follow-through phase, the posterior rotator cuff muscles and scapular stabilizers can absorb the energy [76]. However, throughout a game, these muscles will likely fatigue, thereby reducing the amount of energy that can be absorbed [77]. In this situation, the shoulder will continue to internally rotate to the end range, which will place the remaining force on the posterior capsule. According to Wolf’s law [61], the posterior capsule may adapt to the increased stress by hypertrophying. It has been hypothesized that the posterior capsule will ultimately become thick and fibrotic with repetitive throwing, which will create noncompliant tissue and limit glenohumeral IR. Thomas et al. [68] has measured this thickness with ultrasound and found that the throwing shoulder’s posterior capsule is thicker compared to the nonthrowing shoulder and the thickness correlates with the loss of glenohumeral IR. In addition, several cadaver studies have shown that a tight posterior capsule will shift the center of the humeral head in a posterior-superior direction during the late cocking phase of the throw [78, 79]. A posterior-superior shift of the humeral head has been demonstrated to cause internal impingement and place increased stress at the insertion of the long head of the biceps tendon at the superior labrum [52] (Fig. 1.5). It is expected that the posterior capsule thickens due to throwing; however, it is currently unknown when this adaptation becomes excessive and problematic.
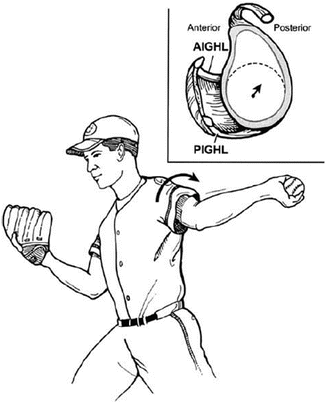
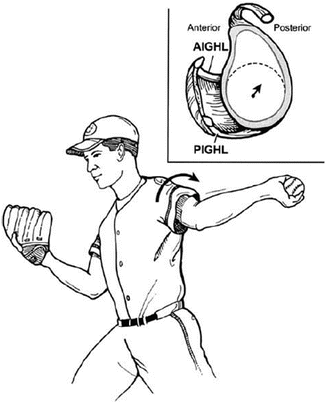
Fig. 1.5
In abduction and external rotation (late cocking), the posterior band of the IGHL is bowstrung beneath the humeral head, causing a posterosuperior shift in the glenohumeral rotation point. Also in late cocking, the biceps vector shifts posteriorly and twists at its base, maximizing peel-back forces. As a result of the tight posteroinferior capsule, this pitcher shows classic derangements of pitching mechanics: hyperexternal rotation, hyperhorizontal abduction (out of the scapular plane), dropped elbow, and premature trunk rotation (Reprinted from Burkhart SS, Morgan CD, Kibler WB. The disabled throwing shoulder: spectrum of pathology Part I: pathoanatomy and biomechanics. Arthroscopy. 2003 Apr;19(4): 404–20, with permission from Elsevier)
The next category of soft tissue that is a concern with throwers is muscle/tendon units. There are several muscle/tendon units that develop similar adaptations at the shoulder girdle. The muscles that will be discussed include the posterior rotator cuff (infraspinatus and teres minor), pectoralis minor, triceps brachii, latissimus dorsi, and the teres major.
First, the infraspinatus and teres minor are the two muscles that comprise the posterior rotator cuff. As stated previously, these two small muscles attempt to repetitively absorb a large amount of the 1.5 times body weight force that occurs during the deceleration phase of the throw [14]. To absorb energy, muscles function eccentrically which entails a forceful breaking of the myosin and actin bonds [80]. Several studies have found that repetitive eccentric contractions of the muscle cause significant damage, often called delayed-onset muscle soreness (DOMS) [81–83]. Several studies have also identified that repetitive eccentric contractions cause an increase in passive stiffness and reduced range of motion that peaks at 24 h and typically takes 4–5 days to return to baseline [84, 85]. It is hypothesized that this clinical presentation is caused by damaging of the sarcoplasmic reticulum, which releases excessive calcium [84]. This has been demonstrated in throwers following a simulated game and is characterized by a decrease in glenohumeral IR immediately following the game [86]. Recently, it has been shown that the loss of IR is still present up to 3 days following pitching [87]. This physiologic phenomenon of muscle would not be a concern if throwing did not occur until after the muscle tissue returned to a normal state. However, most throwers initiate throwing before the muscle tissue returns to normal. Over time, the posterior rotator cuff muscles may develop excessive tightness. The hypothesis is that the immediate loss of glenohumeral IR following throwing will still be present before the next bout of throwing, thereby adding to the total loss of motion. This can continue to occur over the season leading to excessive tightness, characterized by a loss of glenohumeral IR. Posterior rotator cuff tightness is thought to be problematic and is hypothesized that it will alter normal throwing biomechanics [52]. During normal throwing, the shoulder is positioned in the scapular plane (30° anterior to the frontal plane) during the late cocking phase [1, 88]. A tight posterior rotator cuff may position the shoulder in the frontal plane or even in excessive horizontal abduction to release the tension in the posterior rotator cuff. This position will place increased valgus stress on the medial elbow and increased anterior stress on the anterior capsule and cause internal impingement [15, 89]. To address this situation, clinicians should focus on treating the excessive posterior rotator cuff tightness [90–92] and not attempt to change the altered throwing biomechanics. As the muscle tightness is resolved, the altered biomechanics will return to normal.
There are additional muscles that are often overlooked in throwing athletes but do develop significant tightness that can affect throwing biomechanics. The next group of muscles includes the pectoralis minor, triceps brachii, latissimus dorsi, and the teres major. One commonality of these muscles is that they have an attachment on the scapula and therefore can have an effect on scapular motion. The latissimus dorsi is one exception to that rule, but the research isn’t entirely clear. According to most anatomy textbooks, the latissimus dorsi does not have a common attachment site on the scapula, although one cadaver study did recognize that 40 % of the cadavers had an attachment to the tip of the inferior angle [93]. However, the age of the cadavers was not reported, and the attachment may have developed as an adhesion due to patients being bedridden. Future research should identify the prevalence of this attachment in overhead athletes. The pectoralis minor is a thin broad muscle that originates at the third to fifth ribs and inserts on the coracoid process of the scapula. The muscle functions to depress and protract the scapula, although it does not create large ranges of motion. Throwing athletes commonly develop excessive tightness of this muscle with unknown origin [94]. It is thought that the tightness corresponds with inhibition/weakness of the posterior scapular stabilizing muscles, such as the lower trapezius and rhomboids [95]. Since serratus anterior inhibition/weakness is often observed [96, 97] and is the major scapular protractor, pectoralis minor tightness might develop due to compensation for the serratus anterior during throwing. Regardless of the mechanism, pectoralis minor tightness will cause excessive scapular protraction and anterior tilting at rest and during overhead motions [98]. This position has been shown to decrease the subacromial space, which increases the likelihood of developing subacromial and subcoracoid impingement [99]. It also can cause increased anterior stress on the anterior capsule during the late cocking phase of throwing [100]. Stretching is often difficult due to the anatomy of the pectoralis minor, and preventative stretching is likely more effective than waiting until tightness develops [101, 102]. Next, the triceps brachii has a broad origin due to three heads; however, the long head originates on the inferior glenoid and inserts with the other two heads distally on the olecranon process. Therefore, the long head of the triceps brachii is a two-joint muscle and functions at both the elbow and the shoulder. The triceps is very unique since it must coordinate motion at both the shoulder and elbow simultaneously during throwing. This is especially important during the deceleration and follow-through phase of the throw when the muscle is functioning eccentrically to absorb energy. Since the triceps must stabilize and absorb energy at two joints, the stress will be increased within the muscle, thereby creating more eccentric muscle damage during throwing. Similar to the posterior rotator cuff, the triceps will also experience an increase in passive muscle tension and reduced range of motion that requires 4–5 days to recover [85, 103]. Throwing prior to complete recovery will cause the triceps to continually develop tightness throughout the season. Triceps tightness will directly affect scapular motion since its origin is on the inferior glenoid. When the triceps is tight, it has the potential to pull the scapula into excessive protraction and anterior tilting during the deceleration and follow-through phase of throwing. This will cause excessive eccentric loading on the scapular retractors and serratus anterior, which are muscles that often develop inhibition/fatigue. In addition, triceps tightness also has the potential to decrease glenohumeral IR due to the origin on the inferior glenoid. As previously stated, GIRD can be caused from various sources (bone, capsule, posterior rotator cuff, and triceps) and can increase the likelihood of injury [59]. Examining for triceps tightness involves measuring elbow flexed and elbow extended forward flexion with the scapula stabilized [104]. If there is a large increase in forward flexion with the elbow extended, then the loss of motion is caused by triceps tightness. The final group of muscles to be discussed is the internal rotators. Throwing athletes typically have very strong internal rotators [105], which allow them to throw with high velocities. However, the overdevelopment of these muscles often causes excessive tightness. The two major internal rotators that develop adaptive tightness are the latissimus dorsi [106] and the teres major [107]. Both muscles share a common insertion site on the medial aspect of the bicipital groove of the humerus and therefore will affect glenohumeral motion in a similar way when tight. Tightness of these muscles will limit forward flexion and glenohumeral ER. During the late cocking phase of the throw, ER is essential to impart maximal velocity [2, 108]. Typically the thrower is still able to achieve normal ER by compensating at other locations. First, tightness within the latissimus dorsi may cause an excessive lordotic curve within the lower back to ultimately achieve the same glenohumeral ER [31, 106]. Second, tightness within the teres major can directly affect scapular motion [109] to ultimately achieve the same glenohumeral ER, further contributing to the inhibition/weakness of the scapular stabilizers. The tightness can be assessed clinically by examining forward flexion and glenohumeral ER with the scapula stabilized. Stabilizing the scapula is important to isolate the muscular tightness since the scapula can often compensate. The teres major does have a direct attachment on the scapula, making stabilization very important. The latissimus dorsi may have an attachment on the scapula, but it is still debatable. Tightness of the internal rotators has not been greatly recognized in the literature, and therefore, the association with shoulder and elbow injuries is not known.
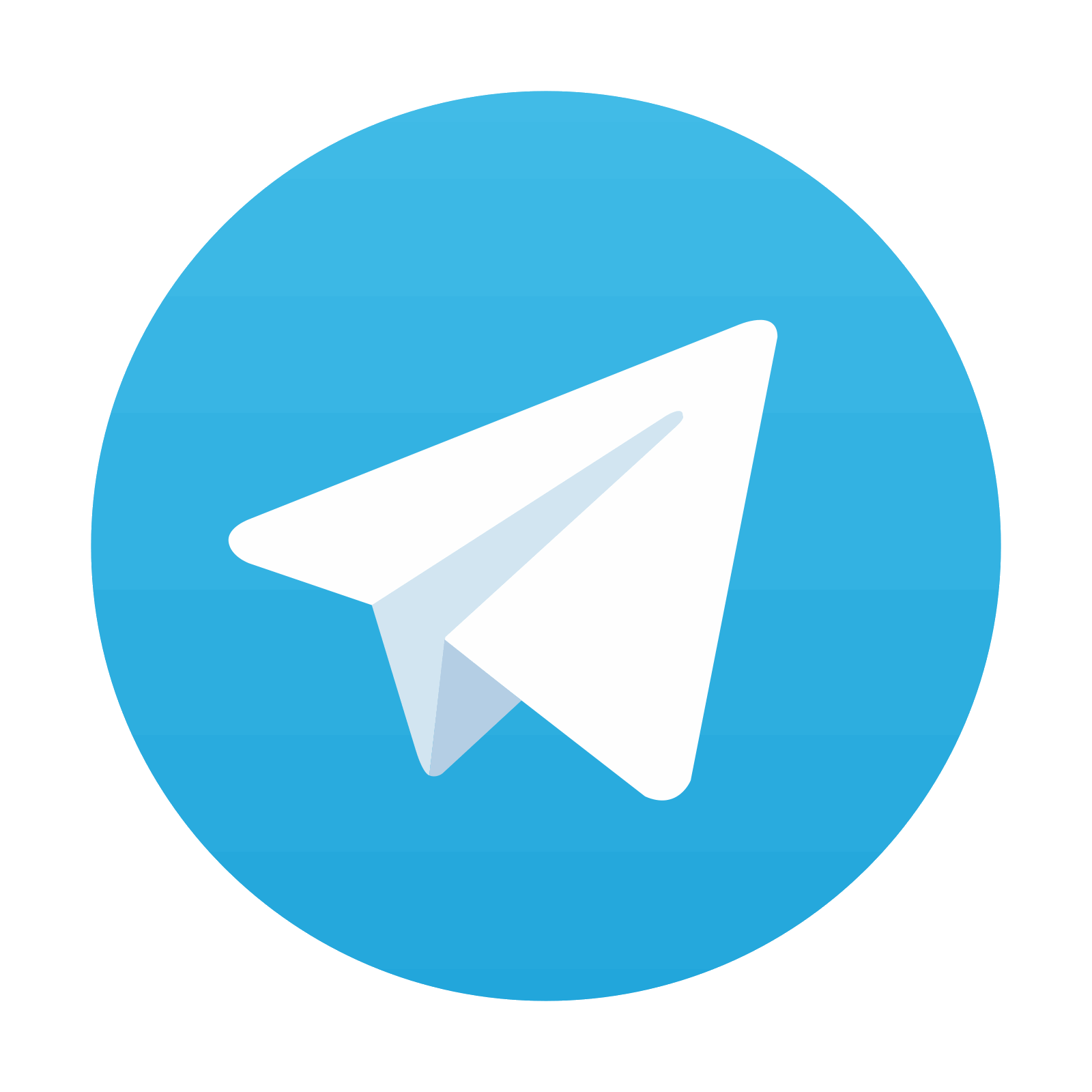
Stay updated, free articles. Join our Telegram channel
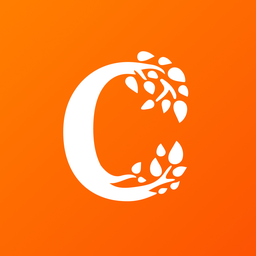
Full access? Get Clinical Tree
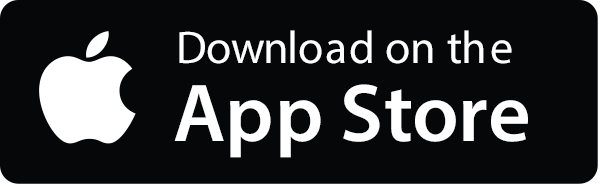
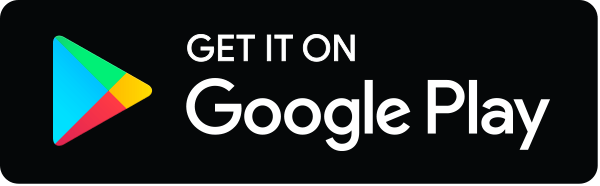