Ambulation requires significant motor coordination. Because of inherent differences in body proportions, level of coordination, motivation, and other factors, each individual gait pattern is unique. However, despite the existence of these individual differences that contribute to each person’s own unique walking style, there are highly repeatable gait sequences, allowing characterization of normal gait patterns and identification of gait disturbance. This article briefly reviews neural control of human ambulation, basic kinematics, and kinetics of normal human gait, and describes the pathophysiology and clinical presentations of spastic hemiparetic and paraparetic, ataxic, and Parkinsonian gait patterns.
Key points
- •
This article reviews normal neural control of human ambulation.
- •
This article reviews basic kinematics and kinetics of normal human gait.
- •
This article describes pathophysiology and clinical presentation of spastic hemiparetic and paraparetic, ataxic, and Parkinsonian gait patterns.
Neural control of normal human ambulation
The neural control required to coordinate the complex motor output of normal human gait is still the topic of significant research. Much of the current understanding is based on animal studies, which have been extrapolated to humans. Animal studies have identified the existence of central pattern generators (CPGs) for ambulation, located in the spinal cord. These CPGs are neural networks that are capable of creating a motor pattern, independent of any sensory input.
The CPG in human locomotion is able to incorporate afferent input from multiple sources to initiate and modify the basic motor pattern of gait and effectively coordinate muscle activation between the 2 lower limbs and between the upper and lower limbs. Voluntary changes in gait, such as change of speed/direction or avoiding an obstacle, seem to be modulated by supraspinal afferents. Reflexive changes in gait, such as stumble recovery, likely involve peripheral sensory afferents of cutaneous and muscle reflexes. There also seems to be differential control of flexor versus extensor muscle groups, with predominant central (supraspinal) control of the flexor muscle groups, and peripheral (proprioceptive) control of the extensor muscle groups.
Many supraspinal structures are involved in the control of ambulation, including the brainstem reticular formation; basal ganglia; motor, premotor, and supplementary motor area of the motor cortex; and cerebellum. The midbrain locomotor region is involved in the initiation of locomotion based on its connections with limbic structures and the basal ganglia. Basal ganglia interactions with the motor cortex via the cerebellum are involved in volitional motor control. Basal ganglia thalamocortical interactions contribute to planning and execution of volitional movement. Known pathways also exist between the basal ganglia and brainstem motor networks, but the precise role of the basal ganglia in controlling muscle tone and locomotion is not well understood. From studies in Parkinson disease (PD), the basal ganglia seem to play a role in regulating step length and the timing of internal cues for each step.
The cerebellum is involved in the coordination of limb movement and overall balance control. The medial cerebellar zone integrates spinal and vestibular inputs (ie, vestibulospinal and reticulospinal tracts), and helps to regulate extensor tone, maintain upright stance and dynamic balance control, and to modulate the rhythmic flexor and extensor muscle activity of locomotion. The intermediate cerebellar zone integrates spinal (dorsal spinocerebellar tract, ventral spinocerebellar tract) and cortical inputs, and helps direct limb placement and regulate agonist-antagonist muscle pairs, playing a key role in controlling the relative timing, amplitude, and trajectory of limb movements, particularly when precision is desired. The lateral cerebellum influences walking via cortical (motor, premotor, somatosensory) interactions and helps make adjustments to the normal locomotor pattern in novel or complex conditions or when strong visual guidance is required.
The most significant sensory afferents for locomotion are from the visual, vestibular, and proprioceptive systems. In addition to modulating the CPG, these afferents also interact with each other. For example, studies have shown that visual inputs decrease muscle spindle activity, and that somatosensory loss can increase sensitivity of the vestibulospinal system.
Proprioceptors located in tendons, muscles, ligaments, and joints relay information regarding limb position and kinesthesia. The dorsal column-medial lemniscus system relays sensory information (localized touch, pressure, vibration, and joint position sense) from the limbs to the sensory cortex. Proprioceptive information transmitted to the cortex assists with controlling volitional movements planned by the motor cortex. The dorsal spinocerebellar tracts relay information from muscle stretch and tension nerve fibers (ie, Golgi tendon organs, muscle spindles) to the cerebellum, and the ventral spinocerebellar tracts relay information from the spinal cord interneurons to the cerebellum. Proprioceptive information transmitted to the cerebellum assists with involuntary modulation of motor control.
Load information carried by mechanical receptors in the sole of the feet and from proprioceptive inputs in the extensor muscles of the foot, and afferents that signal hip-joint position, play a role in leg muscle activation pattern and stance-swing phase transitions during ambulation. For example, excitation of muscle tendon organs in stance contribute to maintaining the stance phase while the limb is loaded, and may also help modulate that activity according to the load carried by the limb.
Neural control of normal human ambulation
The neural control required to coordinate the complex motor output of normal human gait is still the topic of significant research. Much of the current understanding is based on animal studies, which have been extrapolated to humans. Animal studies have identified the existence of central pattern generators (CPGs) for ambulation, located in the spinal cord. These CPGs are neural networks that are capable of creating a motor pattern, independent of any sensory input.
The CPG in human locomotion is able to incorporate afferent input from multiple sources to initiate and modify the basic motor pattern of gait and effectively coordinate muscle activation between the 2 lower limbs and between the upper and lower limbs. Voluntary changes in gait, such as change of speed/direction or avoiding an obstacle, seem to be modulated by supraspinal afferents. Reflexive changes in gait, such as stumble recovery, likely involve peripheral sensory afferents of cutaneous and muscle reflexes. There also seems to be differential control of flexor versus extensor muscle groups, with predominant central (supraspinal) control of the flexor muscle groups, and peripheral (proprioceptive) control of the extensor muscle groups.
Many supraspinal structures are involved in the control of ambulation, including the brainstem reticular formation; basal ganglia; motor, premotor, and supplementary motor area of the motor cortex; and cerebellum. The midbrain locomotor region is involved in the initiation of locomotion based on its connections with limbic structures and the basal ganglia. Basal ganglia interactions with the motor cortex via the cerebellum are involved in volitional motor control. Basal ganglia thalamocortical interactions contribute to planning and execution of volitional movement. Known pathways also exist between the basal ganglia and brainstem motor networks, but the precise role of the basal ganglia in controlling muscle tone and locomotion is not well understood. From studies in Parkinson disease (PD), the basal ganglia seem to play a role in regulating step length and the timing of internal cues for each step.
The cerebellum is involved in the coordination of limb movement and overall balance control. The medial cerebellar zone integrates spinal and vestibular inputs (ie, vestibulospinal and reticulospinal tracts), and helps to regulate extensor tone, maintain upright stance and dynamic balance control, and to modulate the rhythmic flexor and extensor muscle activity of locomotion. The intermediate cerebellar zone integrates spinal (dorsal spinocerebellar tract, ventral spinocerebellar tract) and cortical inputs, and helps direct limb placement and regulate agonist-antagonist muscle pairs, playing a key role in controlling the relative timing, amplitude, and trajectory of limb movements, particularly when precision is desired. The lateral cerebellum influences walking via cortical (motor, premotor, somatosensory) interactions and helps make adjustments to the normal locomotor pattern in novel or complex conditions or when strong visual guidance is required.
The most significant sensory afferents for locomotion are from the visual, vestibular, and proprioceptive systems. In addition to modulating the CPG, these afferents also interact with each other. For example, studies have shown that visual inputs decrease muscle spindle activity, and that somatosensory loss can increase sensitivity of the vestibulospinal system.
Proprioceptors located in tendons, muscles, ligaments, and joints relay information regarding limb position and kinesthesia. The dorsal column-medial lemniscus system relays sensory information (localized touch, pressure, vibration, and joint position sense) from the limbs to the sensory cortex. Proprioceptive information transmitted to the cortex assists with controlling volitional movements planned by the motor cortex. The dorsal spinocerebellar tracts relay information from muscle stretch and tension nerve fibers (ie, Golgi tendon organs, muscle spindles) to the cerebellum, and the ventral spinocerebellar tracts relay information from the spinal cord interneurons to the cerebellum. Proprioceptive information transmitted to the cerebellum assists with involuntary modulation of motor control.
Load information carried by mechanical receptors in the sole of the feet and from proprioceptive inputs in the extensor muscles of the foot, and afferents that signal hip-joint position, play a role in leg muscle activation pattern and stance-swing phase transitions during ambulation. For example, excitation of muscle tendon organs in stance contribute to maintaining the stance phase while the limb is loaded, and may also help modulate that activity according to the load carried by the limb.
Normal human ambulation
Simply stated, the goal of ambulation is to move the body mass from point A to B safely (ie, without falling). This section describes dynamic stability: maintaining upright posture and the body’s center of mass within a moving base of support. When walking, there are periods of double support when both lower limbs are in contact with the ground, and periods of single support when only one limb is in contact with the ground. During single support, the body’s mass moves from being relatively posterior to the supporting limb to anterior, and then alternates being supported by one limb and then the other.
Stance describes the phase in which the lower limb is in contact with the ground. The goals of the stance phase are to maintain stability while supporting body weight and to propel the body forward. Only after weight has been successfully transferred onto the stance limb can the swing phase begin on the contralateral side. Swing describes the phase in which the lower limb is in the air. The goals of the swing phase are to clear the limb over the ground while maintaining forward advancement of the center of mass, and to prepare the limb for the subsequent stance phase. At the basis of ambulation is this phasic cycling of stance and swing, alternating from one lower limb to the other. This basic pattern can be modified in either a proactive or a reflexive manner to meet a particular goal or accommodate differing environmental conditions.
The human gait cycle has been divided into different subphases ( Fig. 1 ).
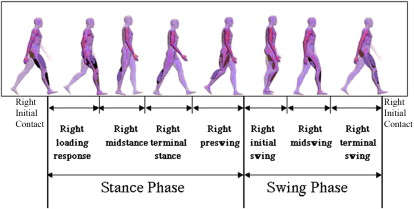
These subphases are useful when observing gait, and particularly when describing the relative timing of an observed deviation or problem. The motion of the key joints of the lower limbs during each of the subphases of gait has also been well described ( Fig. 2 ). Understanding of normal kinematics is useful when describing not only the timing but also the nature of a gait deviation through indicating the problem joint and posture.
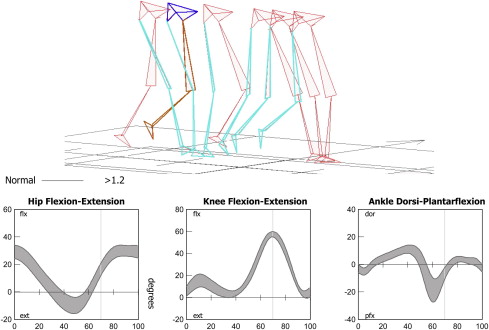
The coordinated patterns of muscle activation during gait are also well described. It is important to recognize that the recording of electromyogram (EMG) activity does not correlate with muscle strength or in some instances even movement, and thus these patterns provide only information about the timing of activation/termination of muscle activity. These activation patterns are phasic in nature, and the timing of activation/termination of activity occurs during well-defined periods of the gait cycle. Abnormalities of muscle activation can include absence of activity, abnormal onset of activation (early or delayed), abnormal duration of activation (abbreviated or prolonged), and presence of abnormal activation pattern (nonphasic pattern, clonic pattern). When both agonist and antagonist muscles are simultaneously activated, this is termed cocontraction . Fig. 3 describes the activation pattern of key muscles during the gait cycle.
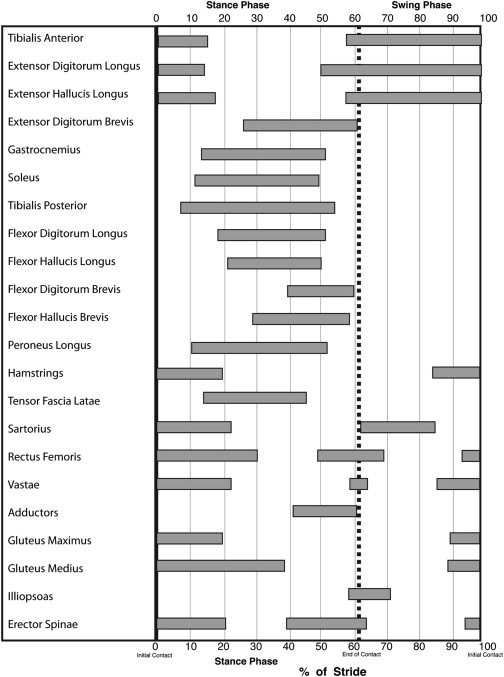
Understanding the functional significance of these muscle activation patterns during gait is one of the most difficult concepts to grasp, most likely because practitioners are anatomically trained to think almost exclusively of muscle activity in terms of concentric muscle contraction patterns in an open chain (eg, gastrocnemius causes ankle plantarflexion, gluteus maximus causes hip extension). This knowledge can be applied in a fairly straightforward manner to the swing phase of the gait cycle. The foot is in the air, and therefore the observed joint movement is caused by active concentric muscle activation. However, the physics of inertia must also be considered. Although the hip flexion observed throughout swing phase is generated by concentric contraction of the hip flexors, the same is not true for the knee flexion observed during swing phase. In fact, the latter is largely passive, an inertial by-product of combined hip flexion and late-stance phase ankle plantarflexion.
During the stance phase, the lower limb is in contact with the ground, forming a closed chain of activation. This mechanism allows muscles to influence joints that they do not cross, thereby increasing the complexity of the relationship between muscle activity and limb movement. For example, when the foot is fixed on the ground, the hip extensors are able to indirectly influence the knee joint, forcing it into extension by pulling the femur posteriorly. When the foot is fixed on the ground, this posterior motion of the femur forces the knee joint into extension passively. Similarly, in a closed chain, the ankle plantarflexors are able to force the knee into extension by pulling the tibia posteriorly. Although one of the roles of the ankle plantarflexors in gait is to generate push-off/propulsion via concentric contraction in late stance, the same muscles also contract isometrically and eccentrically earlier in the stance phase to control forward progression of the tibia, thereby playing a pivotal role in maintaining stance phase stability of the knee joint while minimizing quadriceps activity.
Clinical presentations
Ambulation is the end result of a well-choreographed pattern of phasic muscle activation and deactivation, alternating between 2 lower limbs while transporting the superincumbent mass of the head, trunk, and arms. Given the multiple and complex neural pathways involved in producing ambulation, it is not surprising that disorders of the neurologic system can result in gait disturbance. In patients with neurologic disorders, gait disturbance is a manifestation of a primary problem that alters neural control of ambulation. Because normal pathways are disrupted, alternative means are required, and the net result is an abnormal pattern of locomotion. Importantly, the altered gait pattern that emerges is actually the body’s attempt to achieve the goal of mobility via the use of remaining resources. In the absence of methods to correct the primary neurologic deficit, the goal of clinical gait evaluation and treatment is the optimization of available resources toward the goal of safe and efficient mobility.
Just as normal gait has stereotypic characteristics with individual differences, general patterns of gait disturbance are associated with common central nervous system (CNS) disorders. Obviously, significant variability of gait pattern occurs, even among individuals who share the same neurologic diagnosis; however, general classes of gait dysfunction can still be described, including hemiplegic and paraplegic gait, ataxic, and Parkinsonian gait. These patterns of gait dysfunction can be observed in patients with stroke, traumatic brain injury (TBI), spinal cord injury (SCI), multiple sclerosis (MS), cerebral palsy (CP), and PD, among others.
Spastic Gait
Spastic gait is linked to CNS pathology and is a broad term that is nondescriptive and of minimal significance to the clinician. Spastic gait encompasses hemiparetic patterns (such as associated with cerebrovascular accident, tumor, and TBI), paraparetic patterns (such as associated with SCI, and MS), and gait disorders in patients with CP. This article does not discuss the gait disturbances caused by CP, because they are inherently different due to the presence of a neurologic lesion before onset of normal musculoskeletal and ambulation development. This discussion is limited to adult-acquired hemiparetic and paraparetic gait.
Upper motor neuron (UMN) lesions can impair movement through muscle paresis, problems with muscle overactivity (eg, spasticity, cocontraction, clonus), and increased muscle stiffness. In UMN lesions, even though the CPG for locomotion may be intact, motor control and gait pattern are affected by altered functioning of spinal reflexes and abnormal supraspinal inputs. In addition, the loss of supraspinal drive also has an effect on motor units and muscle function, resulting in a mechanical change in muscle properties. Normally, a relationship exists between muscle activation and development of muscle tension; however, in UMN disorders, this relationship is altered and also contributes to movement disorder.
On physical examination, UMN dysfunction can manifest as hyperreflexia, clonus, and increased muscle tone or spasticity. The finding of muscle weakness on clinical examination may be caused by primary muscle paresis (decreased descending input to motor neurons), decreased ability to recruit or decreased firing rate of volitional motor units, muscle atrophy, change in muscle contractile properties, agonist-antagonist cocontraction with presence of antagonist contraction negatively influencing agonist force generation, or a combination of these.
Dynamic electromyographic studies of lower limb muscles during ambulation can reveal the presence of abnormal muscle activation patterns that reflect problems with timing and coordination of muscle activity, such as absent or reduced amplitude of activation, abbreviated or prolonged activation patterns, premature or delayed activation, loss of phasic activation, and cocontraction of agonist-antagonist muscle groups.
Hemiparetic Gait
Observation of hemiparetic gait pattern reveals an overall marked loss of symmetry with a tendency for increased stance time on the unaffected limb. The normal reciprocal arm motion of gait is absent or diminished on the affected side, and may be flaccid in the early stages of recovery, or positioned in adduction and flexion. The affected lower limb seems stiff-legged, showing an extensor synergy pattern with extension, adduction, internal rotation of the hip; extension of the knee; and plantarflexion/inversion of the foot/ankle. Because of this extended limb posture, individuals have difficulty achieving adequate limb clearance during the swing phase, and observed compensatory maneuvers include hip hiking, lateral trunk sway (away from the affected limb), circumduction, and, less commonly, contralateral vaulting. Initiation of the swing phase is delayed, prolonged, or effortful, and usually associated with stiff-knee posture and ankle equinus ( Fig. 4 ).
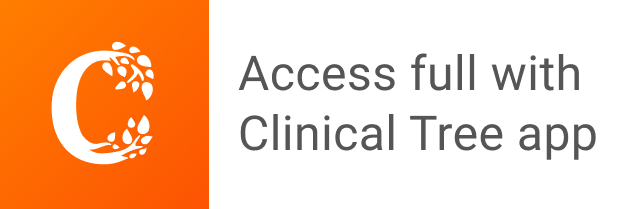