Fig. 1.1
Illustration of the Haversian system and vascular supply in cortical bone. With permission from Springer Science + Business Media: Initiation Fracture Toughness of Human Cortical Bone as a Function of Loading Rate, 2013, C. Allan Gunnarsson
The matrix of bone is approximately 40 % organic and 60 % inorganic [1]. The organic portion of bone is primarily type-1 collagen—the component that provides tensile strength. The remaining organic portion (~10 %) consists of proteoglycans, which provide compressive strength, and matrix proteins. The function of these matrix proteins (e.g., osteocalcin) is to promote mineralization and bone formation. The inorganic component includes calcium hydroxyapatite, which is responsible for compressive strength, and osteocalcium phosphate. The inorganic component is also the mineral portion, which plays a role in calcium metabolic pathways [1].
Normal bone metabolism is a balanced sequence of bone turnover that includes bone breakdown, known as osteoclastogenesis, and bone formation, known as osteoblastogenesis. Osteoclasts are the cells primarily responsible for osteoclastogenesis, and osteoblasts for osteoblastogenesis. Many endogenous hormones regulate metabolism, including parathyroid hormone (PTH), calcitonin, growth hormone, thyroid hormone, estrogen, and testosterone. Endogenous and exogenous steroids, including vitamin D and glucocorticoids, also regulate both calcium and bone metabolism [1]. Factors that promote bone formation do so by either promoting osteoblastogenesis (e.g., PTH, vitamin D) or suppressing osteoclastogenesis (e.g., calcitonin, estrogen). Factors that promote bone breakdown typically suppress osteoblastogenesis (e.g., glucocorticoids).
When stress is applied to bone, Wolff’s law dictates that bone will remodel in response to mechanical stress. The exact method by which bone remodels is not truly understood, but two theories predominate. In the piezoelectric charge theory, tensile-sided strain is said to create electropositive forces that stimulate osteoclastogenesis, while the compression side is subject to electronegative forces that stimulate osteoblastogenesis [1]. The result is the formation or remodeling of bone to increase bone mass on the compressive side in response to mechanical stress. A second theory, the Hueter–Volkmann law, states that bone remodels in small packets of cells in a process called osteoclastic tunneling. Here, there is bone resorption followed by capillaries to introduce blood supply and osteoid-producing cells to lay down new osteoid [1].
Bone Pathophysiology in Stress Fractures
“Stress fracture” constitutes a spectrum of injury that includes bone strain, stress reaction, and stress fracture. The etiology is repetitive loading in the setting of inadequate bone remodeling. The spectrum of injury reflects to some degree the quantity of strain, although exact thresholds are not known and likely mediated by numerous individual host factors in addition to the inciting activity. In general, repetitive injury is more likely to occur in the lower extremity, which sees greater loads than the upper extremity in ambulatory athletes, and with activities that are high volume and offer repetitive loading. Running, for example, produces ground reaction forces approximately five times greater than walking. The result of excess strain is an accumulation of microdamage leading to fatigue reaction or fatigue failure. When the area of fatigue failure is inadequately repaired, it can result in crack initiation in the bone [2] (Fig. 1.2). A simple model is illustrated in Fig. 1.3.
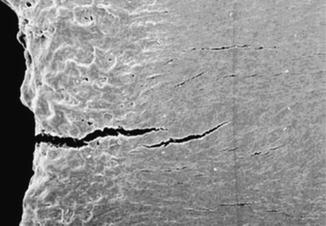

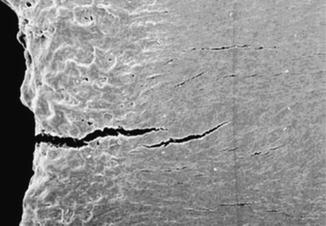
Fig. 1.2
Crack initiation in bone. Reprinted by permission from Macmillan Publishers Ltd: Nature Materials, Nalla RK, Kiney JH, Ritchie O. Mechanistic fracture criteria for the failure of human cortical bone, 2(3). Copyright 2003

Fig. 1.3
A simple model for the propagation of stress injury in bone
Stress injury may also occur with normal strain, but this is typically in the setting of depressed bone remodeling. These injuries are known as insufficiency reactions or fractures. They are more common in the setting of metabolic diseases, hormonal imbalances, and osteoporosis. In the setting of older persons with osteoporosis, both reduced remodeling and structural changes in the trabecular and cortical bone leading to reduced biomechanical strength, and contribute to the susceptibility to insufficiency fracture at physiologic loads [3]. The dichotomy of fatigue failure and insufficiency is certainly more of a continuum with respect to athletes. These individuals experience greater than physiologic strain through activity but also exhibit risk factors for insufficiency failure, putting some subpopulations of athletes at greater risk.
Another special consideration in the pathophysiology of stress fractures in athletes is the influence of skeletal muscle. Muscles may protect the tibia during running by producing shear forces that counteract the joint reaction forces and result in reduced net shear stresses in the tibia. It has been hypothesized that reduced lower leg muscle strength increases the risk of stress fracture through this mechanism [4, 5], and the concept may extend to other common areas of stress fracture. This theory has only been tested in one clinical study, where a significantly lower knee extension power was observed in a case–control study of female runners with and without stress fractures [6]. Others have hypothesized that this potential protective effect of muscle may be diminished with the fatigue associated with excessive training, or be reduced in new exercisers and military recruits [7].
Finally, there is an oxidation deprivation theory of stress fracture development, which deserves some attention. In this theory, the repeated load of an activity such as running is thought to cause decreased oxygen delivery [8] and brief ischemia [9, 10] in weight-bearing bones. This ischemic environment is thought to stimulate the bone-remodeling process, specifically by increasing osteoclastogenesis [11]. The result is weakened bone that is less able to withstand subsequent loads, thereby increasing susceptibility to further stress-related injury. This theory may explain some observations that those new to activity are more at risk [12, 13].
Host Risk Factors for the Development of Bone Stress Injury
Bone Mineral Density and Bone Thickness
Although lower bone mineral density (BMD) is likely a stronger etiological factor in insufficiency fracture development, there is evidence that BMD also plays a role in athletes experiencing fatigue failure-related stress fractures. Loud et al. [14] performed a case–control study of female athletes aged 13–22 years who were diagnosed with their first stress fracture. These patients were matched by age and ethnicity to two controls. The authors demonstrated that cases had lower spine BMD for their age, despite no differences in menstrual irregularity or physical activity participation. Similarly, the odds of a stress fracture were three times that for persons with a family member diagnosed with osteoporosis.
Another case–control study [6] of female athletes aged 18–45 years with and without stress fractures noted that after adjusting for body weight, those with stress fractures had thinner tibial cross-sectional area, lower trabecular BMD, and less cortical area of the posterior tibia.
These associations have been confirmed by prospective studies. The first [15] was a 12-month study of both female and male track and field athletes aged 17–26 years. At baseline, females with lower BMD in the spine were at significantly greater risk of developing a stress fracture. A study of military cadets [16] since has demonstrated that smaller tibial cortical area, lower tibial bone mineral content and smaller femoral neck diameter increased the risk of developing a stress fracture in males, and smaller femoral neck diameter was a risk factor in females.
Genetics
There appears to be some genetic susceptibility to stress fracture. Early investigation concluded that ethnicity was a risk factor for the development of stress fracture, with lower rates seen in African-American compared to Caucasian and Asian women. Much of this difference, however, may be related to inherited differences in bone metabolism through bone mineralization. One study has demonstrated an inherited difference in calcium excretion [17].
The association between a family history of osteoporosis in first degree relatives and increased risk of developing a stress fracture among athletes [6] also suggests there is a genetic role in bone turnover as a risk factor.
Nutritional Factors
Dietary and nutritional factors may play a role in the pathophysiology of stress fracture. Calcium and vitamin D are important components of normal bone metabolism and contribute to BMD, with the former being a mineral building block and the latter playing a role in both calcium homeostasis and bone turnover. One randomized trial of female military recruits found a 20 % reduction in fracture injuries with supplementation of 2,000 mg elemental calcium and 800 IU vitamin D compared to no supplementation [18]. Other research has been inconclusive as to whether dietary intake of calcium is important in the development of stress fractures [19, 20].
Other macronutrients may play a role in susceptibility to stress fractures, although the potential pathophysiologic mechanisms are unclear. Merkel et al. [21] demonstrated that among asymptomatic female military recruits, only those females with low iron anemia developed a stress fracture.
Menstrual Irregularity
Late-onset menarche appears to be a risk factor for stress fracture development [15, 16]. It is unclear whether this is due to low peak bone mass attainment, or whether it is a marker of another influence such as excessive training, or low body weight/body fat. The association is further confounded by the fact that under normal circumstances female athletes appear to reach menarche later than their non-athlete counterparts [22].
Disordered menstruation has also been linked to stress fracture risk. Estrogen functions to increase bone mass by inhibiting osteoclastogenesis. It may also function by reducing the adaptation to stress [23]. As such, numerous studies have demonstrated that female athletes who are amenorrheic [19, 24, 25] or oligomenorrheic [19, 20, 26] are at increased risk of stress fracture. Authors have hypothesized about the combined role of menstrual irregularities and low BMD in some female athletes with the so-called “female athlete triad” (disordered eating, amenorrhea, and decreased BMD).
Summary
Bone stress injury occurs via an imbalance of repetitive stress and normal bone remodeling/recovery in response to that stress. Although the paradigms of fatigue failure (high stress overwhelming normal turnover) and insufficiency failure (normal stress overwhelming disordered turnover) are a simple means of conceptualizing this disorder. In reality components of both will contribute to stress injury in any one individual. This is further complicated when one considers that many of the host factors that influence the pathophysiology of bone stress injury are also interrelated. The findings from a study such as that performed by Cosman et al. [16] illustrate that even with the current state of knowledge, we can explain only a small proportion of the risk for stress fracture development. More research is warranted.
From a practical standpoint, the clinician who will diagnose and treat patients with bone stress injuries must understand the basics of bone biology, including stress remodeling. Once a diagnosis has been made, further probing into the potential role of etiologic factors is recommended. This may include diet and nutritional deficiencies, menstrual irregularity, family history, and training volume. Some of these factors may be modifiable and useful in both the treatment of the current stress injury, as well as the prevention of future injury.
Stress Fracture Epidemiology
The epidemiology of stress fractures is described as the occurrence of stress fractures in athletic populations, and is typically expressed on the basis of exposure (e.g., number of stress fractures per athlete-years or per athlete-exposures). One of the challenges in defining the incidence of stress fractures lies in accurately determining the exposure component. Stress fracture cases are comparatively easy to identify, typically through chart records or physician visits. The challenge of a retrospectively designed study is that while it may identify most or all stress fractures over a given time period, accurate information regarding athletic exposure is comparatively lacking. Consistent and accurate injury reporting data is important to identify risk factors, at-risk subpopulations, and monitor the effectiveness of interventions.
A second complicating factor in deciphering the literature defining the occurrence of stress fractures in athletes is the method of diagnosis. Older studies used modalities such as X-ray, which can have poor sensitivity in identifying changes [27]. Many newer studies utilized bone scan or MRI techniques, which offer greater sensitivity and will identify stress fractures at an earlier stage. The MRI is so sensitive that it can detect stress reaction, a precursor to stress fractures, and thus studies utilizing this method of detection will report a greater incidence/occurrence but for a broader spectrum of clinical disorder. Many of these topics are explored in further detail in the remaining chapters of this text.
This heterogeneity in diagnosis, study design, and accuracy of exposure precludes the pooling of data to formulate incidence rates by sport or activity, at the current time. Therefore, this chapter will focus on a descriptive review of the literature, the most robust of which originates from military populations. Studies from various sports will also be reviewed and interpreted. A preference towards higher level of evidence studies published in the last 10 years is given.
Stress Fracture Epidemiology: Military
Military populations are a unique group that facilitates epidemiological research on stress fractures. Patient follow-up and activity exposure can be well controlled and documented, which allows for more homogeneous comparisons and higher level of evidence designs such as prospective cohorts. Additionally, large numbers of patients can be recruited for study, which is helpful when investigating a condition that typically occurs infrequently or when performing multivariate analyses to identify risk factors. Most important, however, is that military personnel appear to have a higher incidence of stress fractures than the general population due to the suddenly increased and extensive exercise associated with training. Accordingly, military studies on stress fractures have been performed all over the world, including the USA [16, 24, 27–29], Finland [30], and Israel [31].
A common theme in this population is a higher reported occurrence or incidence of stress fractures among females compared to males. In one study of cadets, 19.1 % of females and 5.7 % of males reported at least one stress fracture [16]. Similarly, in the largest studies of US Army recruits [28], the incidence of stress fractures was 79.9/1,000 female and 19.3/1,000 male recruits. This pattern holds true internationally. An Israeli military study [31] identified a similar discrepancy (ratio 2.13) of bone scan positive stress fractures in females (23.9 %) to males (11.2 %). A similar pattern was seen among a prospective cohort of 152,095 Finnish conscripts [30], where the ratio of female to male bone stress injury on MRI was 9:2. The overall incidence rate of stress fractures in this population was 311/100,000 person-years (95 % confidence interval: 277–345).
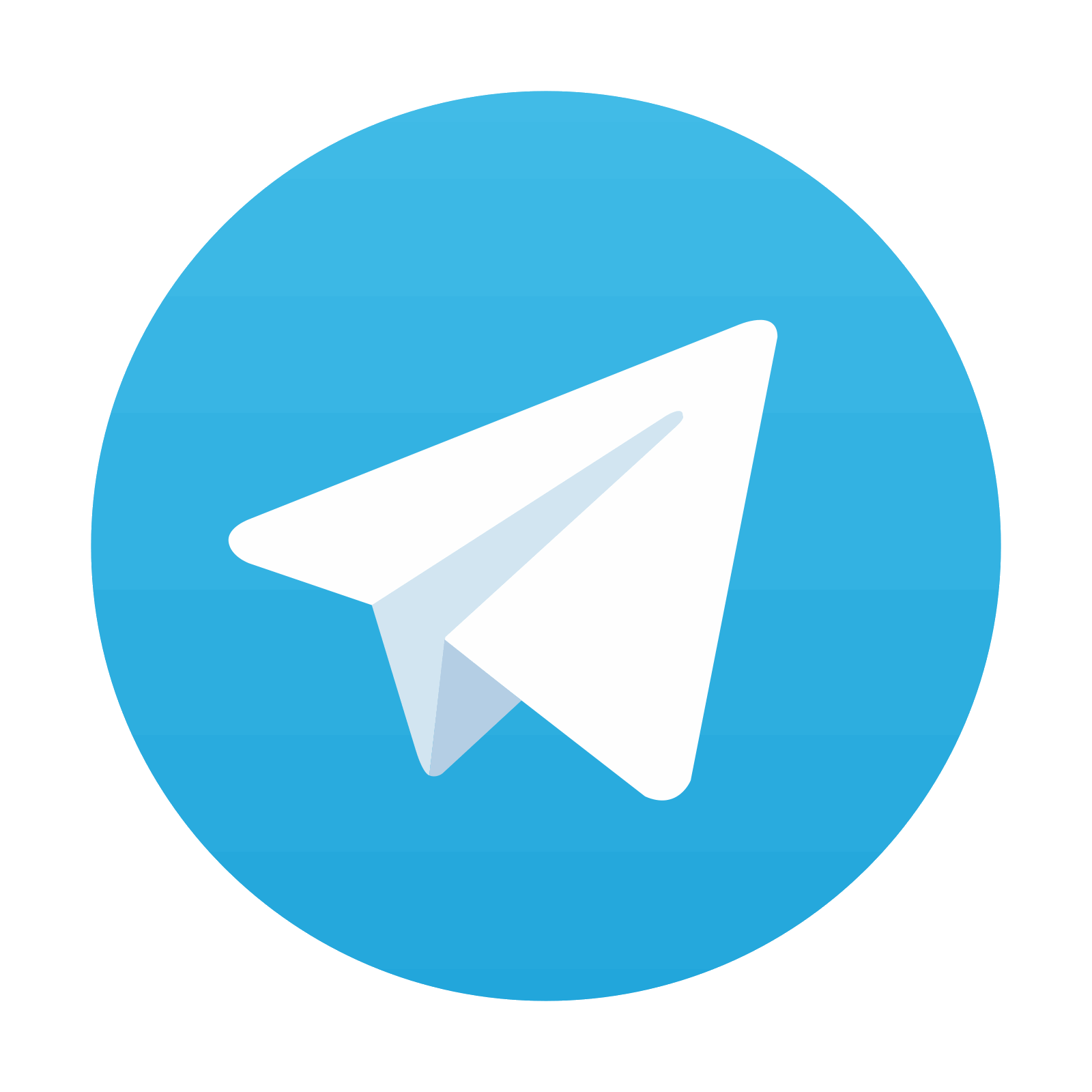
Stay updated, free articles. Join our Telegram channel
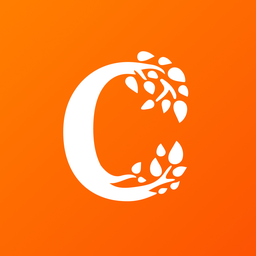
Full access? Get Clinical Tree
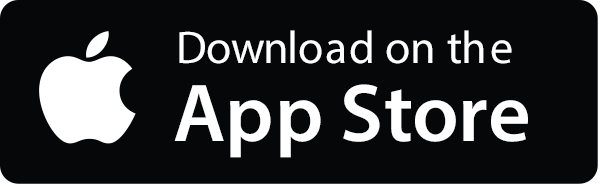
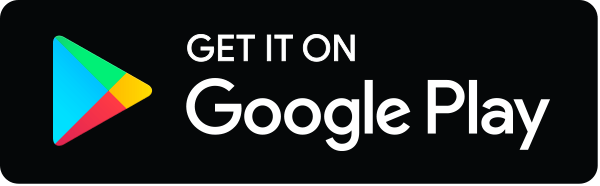