Fig. 5.1
RP. Schematic represention of RP demonstrating cold-induced constriction and vasospasm of the digital arteries, which precipitates a marked reduction in finger blood flow and contributes to the characteristic color changes
Cutaneous Vascular Responses to Cold Exposure in RP
Numerous studies have observed that even in a thermoneutral environment, finger blood flow is lower in RP (primary and secondary) compared to control subjects [19, 22, 31, 58, 60, 78, 125]. During body cooling or a reduction in ambient temperature, blood flow falls to markedly low levels in individuals with RP [19, 31, 58, 78]. Indeed, blood flow can fall to absolute zero in secondary RP (SSc), although this was not observed in individuals with primary RP [31]. In response to localized cooling of the fingers or hands, individuals with primary and secondary forms of RP demonstrate increased sensitivity to cold, with more severe and more prolonged (slower recovery) reductions in finger blood flow [8, 67, 78, 109, 125]. Interestingly, during local warming, finger blood flow was higher in individuals with primary RP compared to control subjects, but remained significantly lower in SSc subjects [147]. This reduced ability of SSc subjects to dilate or increase blood flow is thought to reflect at least in part structural limitations of the SSc circulation (see section “Structural Changes in Cutaneous Circulation”). The ability of local warming to normalize finger blood flow in individuals with RP (including SSc) has been reported by other investigators [67, 109].
Finger blood flow comprises both nutritional blood flow and flow through AVAs (see Chap. 4). In control subjects, total and AVA blood flow are reduced in cool compared to warm environments, but nutritional capillary flow is unaffected and remains constant [19]. In contrast, nutritional, AVA and total flows were all significantly reduced when RP subjects are exposed to a cooler environment [19]. The decrease in nutritional blood flow was most marked for individuals with SSc compared to those with primary RP, whereas the reductions in AVA and total flow were not significantly different between individuals with primary RP and SSc [19]. The increased sensitivity of RP nutritional blood flow to changes in ambient temperature was confirmed by imaging capillaries in the finger nailfold [91] A cool environment did not interrupt nutritional flow in control subjects but had a marked inhibitory effect in individuals with RP [91]. All SSc patients displayed severe disruption in nutritional blood flow, with most presenting a complete “standstill” and others with intermittent “stop and go” pattern of flow [91]. These changes were observed with less severe cooling in RP than was achieved with control subjects. In contrast to SSc patients, individuals with primary RP experienced milder effects on nutritional blood flow, with mostly intermittent standstill of flow [91].
In response to local cooling, digital arteries of individuals with Raynaud’s phenomenon (primary and secondary) constrict more markedly than control subjects, resulting in vasospasm [103, 124, 125] (Fig. 5.2). At a relatively warm skin temperature (35 °C), the diameter of digital arteries was similar between control and RP subjects (luminal diameter of ~1.2 mm) [124]. When finger temperature was gradually reduced, the digital arteries of control and RP individuals initially behaved in a similar manner with slight constriction [124]. However, over the course of a narrow temperature range, digital arteries of RP subjects displayed a dramatic sensitivity to cooling, culminating in vasospasm of RP digital arteries at an average skin temperature of 19.2 ± 0.5 °C (n = 12, mean ± SEM) [124] (Fig. 5.2). In contrast, control arteries demonstrated only a slight constriction in response to cold even when skin temperatures were reduced to 14 °C [124] (Fig. 5.2). Similar results were obtained when the hands of control subjects and individuals with RP were exposed to abrupt local cooling (10 °C), which caused slight constriction of digital arteries in control subjects (8.7 %) but dramatic vasoconstriction in individuals with RP (92.4 %), with no significant difference between those with primary and secondary forms of the disease (including SSc) [103] (Fig. 5.2). Interestingly, local cold-vasospasm of RP digital arteries occurs over a similar temperature range as the local cold-induced closure of AVAs observed in control individuals (see Chap. 4, and section “Endothelial NO” in this Chapter).


Fig. 5.2
Cold-induced vasospasm of digital arteries in RP. The diameters of digital arteries (index or middle fingers) in control subjects and in individuals with RP were determined using ultrasound imaging [103, 124]. Upper panel: Digital arterial diameters were determined in control subjects (+) and in individuals with RP (open square) during progressive local cooling of the finger. Vasospasm was complete in all RP subjects by 17 °C, whereas control subjects displayed only small decreases in diameter. Symbols represent average diameter values [124]. Lower panel: Digital artery diameters were determined in control and RP subjects at room temperature and after local cold stress (hand in 10 °C bath for 5 min) [103]. The cold stress had minimal effects in control subjects, but caused marked constriction in individuals with RP (48 had complete closure) [103]. Closed circles represent individual responses, whereas vertical bars indicate means ± SEM [103] [Images reproduced with permission from [103, 124]]
Mechanisms Contributing to Altered Vasoconstrictor Activity in RP
Sympathetic and Adrenergic Responses
Exposure to a cool environment (body cooling) causes an increase in sympathetic nerve fiber activity to the human cutaneous circulation (Chap. 4). This increase in sympathetic discharge or nerve fiber activity is similar in control subjects and individuals with RP [32]. However, the constrictor response to that sympathetic stimulation [and to the sympathetic neurotransmitter norepinephrine [48]] is increased in RP compared to control individuals, and this increased reactivity is thought to be responsible for the enhanced cold-induced vasoconstriction summarized in section “Cutaneous Vascular Responses to Cold Exposure in RP.”
Increased reactivity of the RP cutaneous circulation to sympathetic stimulation is especially evident in the important regulation of nutritional blood flow. Increased sympathetic activity during body cooling does not impact finger nutritional blood flow in control subjects, but causes a significant reduction in individuals with RP (section “Cutaneous Vascular Responses to Cold Exposure in RP”). This reduction in RP nutritional blood flow was prevented and nutritional flow restored to normal by intra-arterial treatment with low doses of reserpine, which blocks the release of norepinephrine from sympathetic nerve fibers [19]. When control subjects were exposed to the same cool environment, there was no change in nutritional blood flow and no significant effect of inhibiting norepinephrine-induced vasoconstriction on nutritional flow (inhibition of α1-ARs or α2-ARs) [21]. Therefore, sympathetic-mediated constriction expands from predominantly AVAs in normal individuals to additionally encompass the nutritional vasculature in individuals with RP. Indeed, nutritional blood vessels of individuals with secondary RP (SSc) have a marked increase in constrictor activity of smooth muscle α2-ARs, but no change in activity of α1-ARs [36].
The digital artery vasospasm occurring in RP (section “Cutaneous Vascular Responses to Cold Exposure in RP”) is also dependent on sympathetic nerve activity. Reflex increases in sympathetic activity in response to body cooling can precipitate vasospasm in the absence of local cooling, especially in those with secondary disease [12]. However, the vasospastic potential of sympathetic activity in RP is dramatically increased by local finger cooling, and is more marked in individuals with secondary compared to primary forms of the disorder [12]. In contrast, when sympathetic nerve activity is decreased by body warming, the constrictor response to local finger cooling in RP is dramatically reduced [12]. Therefore, vasospasm of digital arteries in RP appears to reflect an increased activity of these blood vessels to sympathetic stimulation and also an increased cold-induced amplification of the response, compared to control subjects [12]. Local cooling of the fingers also amplified sympathetic-mediated constriction of hand blood flow (in response to neck cooling) to a significantly greater degree in RP compared to control subjects, which the authors proposed was mediated by a greater cold-induced amplification of α-AR constriction in RP [67].
Within the local sympathetic process, key mediators of cold-induced amplification are α2-ARs located on the cutaneous smooth muscle cells, which have increased constrictor activity at cool temperatures (Chap. 4). However, assessing smooth muscle constrictor activity of α2-ARs within the intact cardiovascular system is a daunting task. Because of inhibitory prejunctional α2-ARs on vascular sympathetic nerves, α2-AR inhibition will augment sympathetic transmission, increase the release of norepinephrine and promote vasoconstriction (see Chap. 4). Likewise, α2-AR agonists will reduce sympathetic transmission and promote vasodilatation. These responses are the opposite of effects resulting from the inhibition or activation of smooth muscle α2-ARs. This divergence is especially evident following systemic administration of α2-AR ligands. α2-ARs located in the central nervous system powerfully inhibit the sympathetic outflow [15]. Indeed, the preferential α2-AR agonist clonidine was used clinically to treat hypertension, and reduce blood pressure by inhibiting sympathetic activity [110]. Likewise, oral clonidine was evaluated as a potential therapy for RP resulting from hand arm vibration syndrome (HAVS), with the goal of reducing the heightened sympathetic activity in digital arteries [112]. However, clonidine actually provoked RP in some individuals [112]. The observed effects of α2-AR agonists and antagonists will be dependent on the activity of sympathetic nerves, and on whether they are administered locally or systemically. Smooth muscle constrictor activity of α2-AR agonists is best observed when sympathetic activity is low. Likewise, inhibition of sympathetic constriction by α2-AR antagonists will occur only if the response is mediated predominantly, if not exclusively by α2-ARs [39]. The analysis is further complicated by potential deficiencies in the selectivity and activity of the agonists or antagonists. For example, clonidine is a partial agonist with weak activity at α2-ARs and very weak activity at α2C-ARs [36]. Where possible, high efficacy agonists should be used (e.g., brimonidine—UK14,304) [36].
Local selective inhibition of α2-ARs abolished cold-induced vasospastic attacks in individuals with primary RP, whereas inhibition of α1-ARs had no effect [45]. In primary RP, the low finger blood flow during exposure to a cool environment was dramatically increased by local selective inhibition of α2-ARs (sevenfold) or combined non-selective inhibition of α1-ARs and α2-ARs (sixfold), whereas local selective inhibition of α1-ARs had a much smaller effect (twofold) [20]. In a separate study, under thermoneutral conditions, α1-AR inhibition had a greater effect to increase blood flow in controls compared to primary RP, such that the difference in blood flow between controls and RP actually increased [22]. In contrast, α2-AR inhibition caused greater dilatation in RP compared to controls and therefore diminished the differences in blood flow between these groups [22]. In individuals with primary RP, inhibition of α1-ARs or α2-ARs prevented vasoconstriction in finger blood flow to moderate local cold exposure [22]. This contrasts with other studies where α1-AR blockade did not prevent cold-induced vasoconstriction in human finger blood flow in normal or RP subjects [18, 30]. In individuals with secondary RP (HAVS), cold-induced vasoconstriction was abolished after local selective inhibition of α2-ARs [85].
Molecular expression of α-ARs on RP cutaneous arteries has not been determined. However, expression of α2-ARs on circulating platelets is increased in individuals with primary RP compared to controls [28, 69]. Furthermore, the constrictor activity of smooth muscle α2-ARs is dramatically and selectively increased in RP (SSc) compared to control arteries, which would be consistent with increased receptor expression [36] (Fig. 5.3). Similarly, when administered intra-arterially in a thermoneutral setting, the preferential α2-AR agonist clonidine caused vasoconstriction in human fingers that was significantly increased in primary RP compared to control subjects [20] (Fig. 5.3). In contrast, constriction to the preferential α1-AR agonist phenylephrine was similar between the two groups [20] (Fig. 5.3). Other studies confirmed this increased responsiveness to clonidine in primary RP compared to control subjects, although they also observed increased responsiveness to phenylephrine [49, 50]. When assessed during body heating (to reduce sympathetic activity), local cooling increased the constriction of finger blood flow to the preferential α2-AR agonist clonidine in individuals with primary RP, whereas in control subjects local cooling actually inhibited responses to the agonist [49]. Local cooling did not affect constriction to the preferential α1-AR agonist phenylephrine in controls or RP subjects [49]. In contrast to these analyses of finger blood flow, when constrictor activity was assessed directly in non-glabrous skin, norepinephrine or the α2-AR agonist BHT933 evoked cutaneous vasoconstriction that was similar in control and secondary RP individuals (HAVS), whereas phenylephrine caused constriction that was reduced in RP (HAVS) [29].


Fig. 5.3
α2-AR vasoconstriction is selectively increased in RP. Left panels: Fingertip blood flow was assessed in control subjects (open square) and in individuals with primary RP (closed square), under basal conditions and in response to increasing intra-arterial doses of the preferential α2-AR agonist clonidine (top) or the preferential α1-AR agonist phenylephrine (bottom) [20]. Constrictor responses to clonidine were increased in RP compared to controls, whereas responses to phenylephrine were similar in control and RP individuals [20]. Right panels: Contractile responses of nutritional arterioles isolated from control subjects (open circle) and individuals with secondary RP (SSc, closed circle) were assessed in response to the selective α2-AR agonist UK 14,304 (top) or the α1-AR agonist phenylephrine (bottom). In addition, the response to UK 14,304 is also presented for endothelium-denuded arterioles (open and closed squares). SSc arterioles had increased reactivity to α2-AR stimulation, whereas responses to α1-AR were not significantly different between control and SSc arterioles
Despite the caveats surrounding analysis of smooth muscle α2-ARs, the ability of selective α2-AR antagonists to prevent the vasospastic attacks of RP and to inhibit cold-induced vasoconstriction of finger blood flow in RP strongly implicates increased activity or expression of smooth muscle α2-ARs in the pathogenesis of this disorder. This conclusion is reinforced by the increased constrictor activity of the preferential α2-AR agonist clonidine in individuals with primary RP and increased contractile response to the highly selective α2-AR agonist UK14,304 in secondary RP arterioles (SSc). Increased activity of α2-ARs would explain the increased constrictor activity of the sympathetic nervous system (e.g., in response to body cooling), and the increased cold-induced amplification of that constriction in RP (Fig. 5.4). A key component of RP pathogenesis is the expansion of powerful sympathetic-mediated vasoconstriction from predominantly AVAs in control subjects to digital arteries and arterioles in individuals with RP (Fig. 5.5). This expansion of sympathetic constriction, which is also likely to be mediated by increased activity of smooth muscle α2-ARs, explains the interruption in nutritional blood flow that occurs in primary RP and to a greater degree in secondary RP (SSc) (Fig. 5.5) (see also section “Endothelial NO”).








Fig. 5.4
Schematic representation of sympathetic neurotransmission in cutaneous blood vessels and its likely modulation in RP. Norepinephrine (NE) is located in small storage vesicles within sympathetic nerve varicosities. (a): Controls. During Body Cooling, there is increased activation of sympathetic nerve fibers and exocytotic release of NE, which causes blood vessel constriction by predominantly stimulating α2-ARs located on the smooth muscle cells. Norepinephrine also activates prejunctional α2-ARs located on the sympathetic nerves to inhibit release of sympathetic neurotransmitters. Local cooling amplifies α2-AR constrictor activity, but inhibits smooth muscle constriction and α1-AR-mediated responses. Smooth muscle α-ARs can also be activated by circulating norepinephrine and epinephrine (Epi) released from the adrenal medulla (and other sympathetic nerves). The endothelium releases NO, which causes dilatation of smooth muscle cells. Local cooling is thought to reduce this dilatation, which would result in amplified α2-AR constrictor activity. (b): Primary RP. Increased activity of α2-ARs on smooth muscle cells is likely responsible for the increased constriction to sympathetic nerve stimulation (including during Body Cooling) and to increased amplification of sympathetic constriction in response to local cooling. (c): SSc. SSc is additionally characterized by a marked change in endothelial function with reduced activity of NO, precipitating increased constrictor responses to α2-AR stimulation and increased exocytosis of ET-1 and ULVWF (VW). This latter response appears to be most evident in superficial blood vessels and the resulting constriction to ET-1 is likely most prominent in nutritional arterioles. SSc arteries are also associated with intimal fibrotic lesions mediated by mesenchymal cells. Solid arrows indicate activation, whereas hatched arrows reflect inhibition


Fig. 5.5
Schematic representation of the cutaneous vascular system in human glabrous skin in control (a), primary RP (b), and SSc (c) subjects. The arterial supply system branches to an arteriovenous anastomosis (AVA) and also to nutritional capillary loops. (a): Controls. In response to Body Cooling, there is increased activity of sympathetic nerves, which release norepinephrine to cause constriction predominantly of the AVA structures by activating α2-ARs located on the smooth muscle cells. AVAs appear to have increased activity of this constrictor mechanism and nutritional blood flow through capillary loops is protected from sympathetic constriction and is therefore maintained. Local cooling amplifies sympathetic α2-AR dependent constriction of AVAs. (b): Primary RP. In Primary RP, Body Cooling causes the same increase in activity of sympathetic nerves, but cutaneous blood vessels have a more pronounced response to the sympathetic neurotransmitter(s). This likely represents increased expression of smooth muscle α2-ARs causing increased constriction of cutaneous veins, AVAs and the arterial supply. Increased expression of constrictor α2-ARs would also cause these blood vessels to be more responsive to local cooling. This expansion of sympathetic and local cold-induced constriction to more proximal arteries and to nutritional arterioles is responsible for the vasospasm of digital arteries and for slight disruption in nutritional blood flow to capillary loops. (c): SSc. In SSc, in addition to increased sympathetic constriction, there are structural changes in the vascular system comprising intimal lesions in arteries and arterioles and disruption of the nutritional capillaries. Because of these changes and the additional dysfunction in arterial endothelial flow-mediated dilatation, the profound constriction occurring in the upstream arterial system causes profound disruption in nutritional blood flow and ischemic injury
Vascular Mechanisms Underlying the Characteristic Color Changes of RP
RP episodes are generally associated with progressive color changes: initial pallor or blanching followed by cyanosis then rubor, which has been likened to the French tricolor of white, blue and red [74] (Fig. 5.1). The initial pallor of affected skin reflects cold-induced digital artery vasospasm, which will cause an abrupt and marked reduction in arterial inflow. However, the remarkable whitening suggests that cutaneous veins also undergo severe cold-induced vasoconstriction (venous spasm), which would rapidly remove blood from the low pressure, large volume venous system (see Chap. 4 for discussion). Veins are generally considered as weak and sluggish effectors. Although this is often the case in deep veins, cutaneous veins exert very powerful and rapid responses to sympathetic activation [39, 41]. Furthermore, sympathetic neurotransmission can actually be more effective in cutaneous veins compared to arteries. In arteries, because of their high pressure, nerve fibers are restricted to the outer circumference of the blood vessel wall, and norepinephrine diffuses between smooth muscle cells to regulate vascular contractility and diameter (Fig. 5.4). In contrast, in the low pressure cutaneous venous system, sympathetic nerve fibers penetrate the blood vessel wall and release norepinephrine directly on smooth muscle cells. The smooth muscle of cutaneous veins also has an exceptionally high activity of α2-ARs, making them highly responsive to local cold-induced amplification of sympathetic constriction [17, 37, 42].
The initial pallor of the skin can be followed by the cyanotic phase (Fig. 5.1). This is often ascribed to hypoxia or lack of oxygen causing inappropriate deoxygenation (and hence blueing) of the blood. However, it is likely mediated by early vasodilatation of the cutaneous venous system while the AVAs remain in a highly constricted state. The dilated cutaneous venous system would therefore expand with deoxygenated blood flowing from nutritional capillaries and from deeper veins. The venous system in glabrous skin likely has a high volume or capacity, which would be required to accommodate high levels of AVA blood flow during heat stress. Therefore, the dilated cutaneous venous system will provide a high capacity for deoxygenated blood and also a low flow rate, resulting in the unusual cyanotic appearance. More rapid dilatation of cutaneous veins compared to AVA could reflect the more intimate association of sympathetic nerves with venous smooth muscle, which would allow for more rapid removal of norepinephrine (via neuronal uptake), or from differences in smooth muscle contractile dynamics in veins versus AVAs.
The cyanotic phase is followed by rubor or reddening of the skin. This phase is often attributed to ischemic dilatation of the skin’s nutritional blood supply (hyperemia). However, a more likely explanation is that it predominantly reflects the delayed dilatation of AVAs allowing a large influx of fully oxygenated blood (AVA flow bypasses the nutritional capillaries) into the dilated venous system. The high flow of this richer red blood through the dilated venous system will displace the bluer deoxygenated blood and provide the rubor appearance of the skin.
Finally, normal sympathetic regulation of the cutaneous arterial system, the AVAs and venous system will restore normal coloration to the skin.
Endothelial NO
Endothelial cells respond to numerous stimuli by increasing production of the powerful vasodilator and protective mediator NO, as well as secondary vasodilators such as prostacyclin (Chap. 4) (Fig. 5.4). Indeed, even norepinephrine acting on endothelial α2-ARs can initiate endothelium-dependent vasodilatation [40]. Although endothelial α2-ARs are most active in the coronary circulation, they have the potential to initiate dilatation in cutaneous arteries [23, 40]. The physical action of the blood stream is a key endothelial activator, with increasing blood flow causing increased production of endothelial dilators and endothelium-dependent dilatation [26, 44].
Endothelial NO activity is generally reduced in vascular disease resulting in a diminution in its protective activity and precipitating pathological functional and structural changes in the blood vessel wall, including enhanced constriction, vascular cell death (apoptosis), vascular inflammation, and vascular remodeling [34, 43, 84, 140]. Most studies have found that endothelium-dependent dilatation is normal in individuals with primary RP, including in response to increased flow (flow-mediated dilatation) [2, 73, 88, 114]. However, some reports have reported increased and decreased endothelial responses [71, 72], although this variation could reflect in part differences in gender and/or age between the experimental groups. In individuals with RP secondary to SSc, studies have consistently demonstrated impaired vasodilatation to endothelial activators including flow-mediated vasodilatation, and that this dysfunction occurs early and worsens as the disease progresses [1, 2, 46, 47, 80, 82, 83, 88, 93, 116, 117] (Fig. 5.4). Vasodilatation to endothelium-independent, direct smooth muscle dilators (NO-mimics: nitrovasodilators) may be preserved [46, 47, 128] or reduced in SSc [2, 80, 82, 83, 93, 117]. Therefore, the decrease in SSc endothelial dilatation may progress from dysfunction localized to the endothelium, to pathological changes throughout the blood vessel wall including structural changes that limit vasodilatation. These pathological changes in the SSc cutaneous circulation are part of a widespread systemic vasculopathy [4, 6, 25, 132, 133] (section “Structural Changes in Cutaneous Circulation”) (Fig. 5.4).
Endothelial dilatation is likely to have an especially important role in glabrous skin including the finger circulation, where high blood flow through AVAs will expose proximal digital arteries to high shear stress and endothelial activation. Interestingly, RP is restricted to areas that are rich in AVA, with digital artery vasospasm occurring over a similar temperature range as cold-induced closure of AVAs (see section “Cutaneous Vascular Responses to Cold Exposure in RP”). The closure of AVAs, by reducing shear stress-induced activation of digital artery endothelium, may shift the balance towards sympathetic vasoconstrictor activity. Although diminution in shear stress-induced endothelial activity would also occur in control subjects, they would continue to be protected from vasospasm by a reduced sympathetic stimulus for digital artery constriction [12, 141] (Fig. 5.5).
Alterations in endothelial-dependent dilatation also likely contribute to the differing influence of cold exposure on nutritional blood flow in primary RP and secondary RP (SSc). As discussed above, during cold exposure, nutritional blood flow is markedly disrupted in secondary RP (SSc) compared to the minor impairment observed in primary RP. Endothelial flow-mediated vasodilatation enables dilation in distal compartments of the circulation (e.g., terminal arterioles) to be conducted upstream and provide graded and targeted increases in blood flow (see Chap. 4). Therefore, despite the remarkable whitening in primary RP, metabolic vasodilation (Chap. 4) in nutritional arterioles will provide an endothelial dilator stimulus to maintain nutritional blood flow (albeit somewhat restricted because of profound arterial constriction). This low-level blood flow will quickly exit the constricted venous system and therefore not influence the pallor of the skin (see section “Vascular Mechanisms Underlying the Characteristic Color Changes of RP”). In contrast to primary RP, there is severe endothelial dysfunction in SSc. Therefore, although metabolic activity may dilate the local SSc nutritional arterioles, reduced flow-mediated dilatation will prevent this dilation from being conducted upstream to proximal arteries, which will continue to markedly restrict blood flow. Therefore, unlike primary RP, there will be a more profound interruption in SSc nutritional blood flow, precipitating SSc tissue injury.
A reduction in endothelial NO activity would also be expected to amplify vasoconstrictor episodes in SSc arteries, and to promote further pathological changes in the vascular system including thrombotic and inflammatory signaling and vascular remodeling. Indeed, expression of cold-sensitive α2C-ARs in human cutaneous smooth muscle cells is amplified by inflammatory stress [15–17].
Endothelin-1 and Endothelial Exocytosis
Endothelin-1 (ET1) is a powerful vasoconstrictor, inflammatory and fibrotic mediator that can be produced and stored (as its precursor, Big ET1) within the vascular endothelium [56]. Following appropriate stimulation, endothelial cells quickly release stored proteins including Big ET1 by exocytosis [56]. Big ET1 is rapidly converted to ET1 during this exocytotic process [56]. There has been considerable interest in the potential role of ET1 in contributing to the vasospastic attacks of RP and to pathological vascular and tissue remodeling in SSc. Indeed, endothelial exocytosis can apparently be activated by exposure to cool temperatures [152]. However, under normal physiological conditions, the endothelium neither synthesizes nor releases sufficient quantities of ET1 to initiate vasoconstriction [56]. Furthermore, NO is a powerful endogenous inhibitor of ET1 expression and of endothelial exocytosis [56]. Although early research suggested that circulating ET1 levels were higher in individuals with primary RP compared to controls, and were further increased by local cold exposure [153], subsequent studies revealed conflicting results and ET1 is not thought to be involved in the vasospastic episodes of RP [27, 125, 146].
Endothelial exocytosis mediates the release of other stored proteins including ULVWF (ultra large von Willebrand factor), which promotes hemostasis and thrombosis [13, 56]. ULVWF is unfurled by blood flow and is subsequently cleaved by an endothelial surface protease ADAMTS13 to generate (circulating) VWF fragments with reduced thrombotic potential [13]. Release of ULVWF occurs in parallel with the release/generation of ET1 [56]. In controls and in individuals with primary RP, there is minimal evidence for endothelial exocytosis of ULVWF in cutaneous blood vessels [75]. In contrast, there is exuberant release of ULVWF in SSc superficial skin blood vessels with concomitant loss of ULVWF storage in the associated endothelium [75] (Fig. 5.4). The extent of endothelial exocytosis is related to SSc disease progression [75]. A similar pattern is evident with the circulating level of VWF, which is normal in primary RP but significantly increased in individuals with SSc and correlates with disease severity [9, 57, 64, 89, 90]. The circulating level of VWF is not influenced by cold exposure [9]. Increased exocytosis of ULWF, which will be a powerful stimulus for thrombosis and vascular inflammation, may reflect diminished activity of NO in SSc (Fig. 5.4). The contents of endothelial storage granules can be regulated to increase expression of pathological mediators, including Big ET1. Indeed, the expression and storage of Big ET1 is dramatically increased in vascular aging [56]. There is increased presence of ET1 in cutaneous blood vessels of SSc subjects compared to control individuals, and as occurred with ULVWF release, ET1 is localized predominantly to superficial blood vessels [138]. Therefore, as occurs during aging, SSc endothelium likely has increased expression of ET1 precursors, with ET1 being formed during endothelial exocytosis (Fig. 5.4). Indeed, individuals with secondary RP (including SSc) have markedly increased circulating levels of both VWF and ET1, with close correlation between them [119, 139]. Short-term treatment with bosentan, which antagonizes ETA (mediates smooth muscle constriction) and ETB receptors (mediates endothelial generation of NO, and sometimes smooth muscle constriction), has shown promise in significantly reducing the formation of new ulcers in SSc subjects, although it does not significantly influence vasospastic attacks of RP [76, 92, 106]. This may reflect an increased role of ET1 in the nutritional microcirculation rather than in proximal digital arteries, which is consistent with the increased prominence of endothelial exocytosis in more superficial blood vessels.
There have been promising preliminary results obtained with botulinum toxin A (botox) in treating severe RP (including SSc), reportedly improving pain, perfusion and ischemic lesions [51, 104, 105, 121, 135, 137]. It remains unclear how this agent might mediate beneficial effects in this disease spectrum. Botulinum toxins specifically inhibit the molecular machinery involved in exocytosis, targeting macromolecular “SNARE” complexes involved in vesicle fusion with the plasma membrane [97, 144]. They can therefore inhibit the exocytotic release of neurotransmitters from nerve fibers. Botulinum toxin A comprises a heavy chain (Hc) and light chain (Lc), with the Hc involved in the binding and internalization of the toxin and the Lc subsequently degrading the SNARE protein SNAP25 [97, 144]. Cell surface receptors for the toxin comprise high affinity proteins (SV2, synaptic vesicle protein 2) and low affinity glycolipids called gangliosides [97, 144]. The toxin is thought to have preferential activity to inhibit cholinergic neurotransmission (including the neuromuscular junction in skeletal muscle) because of a higher expression of SV2 receptors [97, 144]. Indeed, when administered in low doses to human non-glabrous skin (forearm), botulinum toxin A selectively inhibited the vasodilatation and sudomotor (sweating) responses caused by heat stress-induced activation of the sympathetic cholinergic system [70, 122] (see Chap. 4 for description). In contrast, it had no effect on the sympathetic adrenergic cutaneous vasoconstriction caused by body cooling or the neuropeptide-mediated vasodilation evoked by local warming [70, 122]. However, when used in higher concentrations, the toxin can access sympathetic nerves to cause proteolytic degradation of SNAP25 and inhibition of sympathetic neurotransmission [98, 99, 126]. Indeed, botulinum toxin A also powerfully inhibits endothelial exocytosis [108], and will therefore inhibit the release of ULVWF and ET1. Although cold-induced translocation of smooth muscle α2-ARs involves fusion of transport vesicles with the plasma membrane [100], it is not clear if this pathway involves SNARE complexes. The potential therapeutic effects of botulinum toxin A will therefore be determined by the dose and route of administration, but also the ability of the toxin to access target cells and the role of SNAP25 in mediating vesicle fusion in those cells. Based on available data, the most likely therapeutic targets include sympathetic-mediated vasoconstriction and endothelial exocytosis of ET1 and ULVWF. Endothelial production of NO is not dependent on exocytosis and should not be influenced by the toxin. However, the toxin may have a negative impact on responses to body warming (cholinergic dilatation, sweating) and local skin warming (neuropeptide dilatation).
Others
Although the vasospastic episodes of RP reflect abnormal thermoregulatory responses, we lack insight into thermoregulation in these individuals. During cold exposure, processes are initiated to restrict heat loss (cutaneous vasoconstriction) and to generate heat (thermogenesis) (see Chap. 4). Analysis of the RP population has so far been restricted to the process of cutaneous vasoconstriction and reduction in heat loss, with no studies assessing thermogenesis (including shivering and activation of brown adipose tissue, BAT). Activation of thermogenesis has the potential to diminish the role of heat conservation and cutaneous vasoconstrictor mechanisms. Indeed, if perivascular adipose tissue (PVAT, Chap. 4) of cutaneous blood vessels can participate in thermogenesis, then such local heat production could diminish the influence of local cold exposure on cutaneous vasoconstrictor mechanisms. Despite the exuberant cold-induced cutaneous vasoconstriction in RP, core temperature was actually lower in RP in a thermoneutral environment and fell more during a reduction in ambient temperature, when compared to control subjects [58, 59]. However, core temperatures of control and RP individuals were similar in a warm environment [58]. This preliminary analysis suggests that thermogenic responses may be impaired in RP.
The density of CGRP-containing nerve fibers in finger skin (non-glabrous) is reported to be reduced in individuals with primary RP and further decreased in SSc [11]. Although CGRP is a vasodilator and has specific efficacy in terminating ET1 activity [81, 94, 95], no studies have provided evidence that a deficiency in this mediator contributes to heightened vasoconstriction in RP. As discussed in Chap. 4, activation of CGRP-containing nerve fibers appears to be involved in the immediate cutaneous vasodilatation to local warming [68]. However, cutaneous blood vessels of RP subjects dilate more to local warming than control subjects, with SSc subjects displaying an intermediate or reduced response [141, 147]. Therefore, there appears to be no functional deficit in the neuropeptide response to warming in primary RP, and the dysfunction present in SSc may represent a structural limitation of the SSc vasculature (see section “Structural Changes in Cutaneous Circulation”).
Increased activity of reactive oxygen species (ROS) may play an important role in acute vasospastic episodes of RP and in the vascular and tissue remodeling occurring in SSc [1, 62, 146]. Increased ROS activity contributes to cold-induced cutaneous vasoconstriction by stimulating smooth muscle α2C-AR mobilization and potentially by inhibiting NO dilatation, impairs the protective role of the endothelium, and participates in vascular and tissue fibrosis [5, 35, 62, 146].
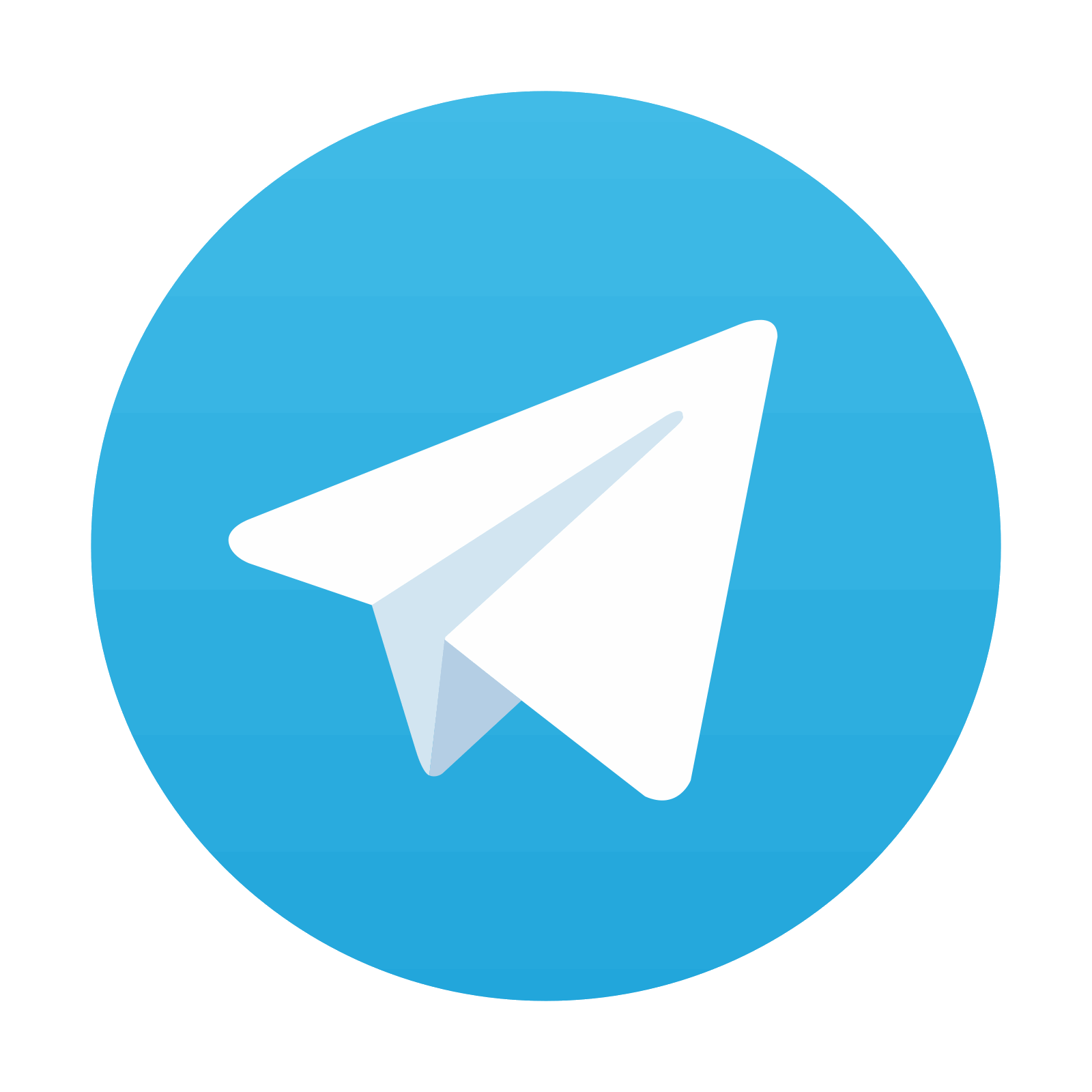
Stay updated, free articles. Join our Telegram channel
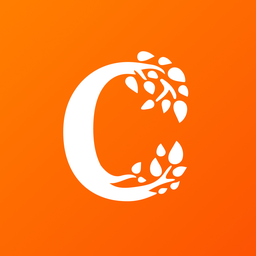
Full access? Get Clinical Tree
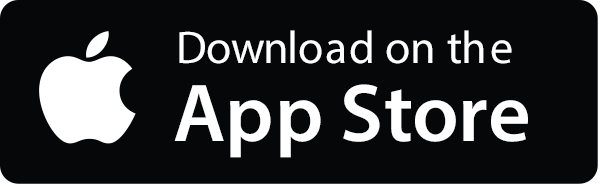
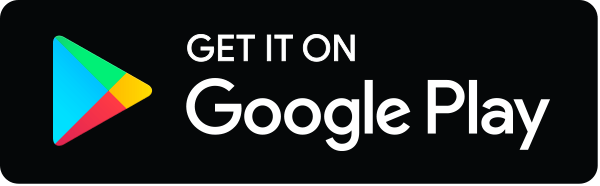