Fig. 2.1
Normal bone remodeling
Osteoblasts
Osteoblasts differentiate from mesenchymal stem cells located in the bone marrow stroma. They regulate bone mineralization and synthesize the dense cross-linked collagen that will form the bone matrix. Essential for osteoblast differentiation is the transcription factor RUNX2, or core binding factor A1 (CBFA1). Mice lacking RUNX2 show arrest in osteoblast maturation and, therefore, do not develop bone [10, 11]. Several systemic and local factors produced by osteoblasts play an important role in bone metabolism. Some of these factors are prostaglandins, receptors for PTH, estrogen, vitamin D3, and several cytokines, such as TGFβ, PDGF, and fibroblast growth factor (FGF) [12, 13]. Osteoblasts hold a very important function in regulating osteoclast formation and differentiation, stimulating it through the expression on their cell surface of the receptor activator of nuclear factor kappa B (RANK) ligand (RANKL) , which interacts with its cognate receptor, RANK, expressed in the osteoclast precursor membrane. Osteoblasts can also inhibit osteoclast differentiation by the secretion of osteoprotegerin (OPG), a soluble RANK receptor, which functions as RANKL antagonist.
A major regulator of osteoblast differentiation and function is the Wnt pathway [9]. The activation of Wnt/β-catenin signaling results in increased bone mass, and overexpression of Wnt10 in animal models also leads to increased bone mass. In osteoblastic precursor cells, overexpression of Wnt7B and β-catenin induces differentiation of these cells into mature osteoblasts [14, 15]. Evidence indicates that both canonical and noncanonical Wnt signaling pathways are implicated in mediating these effects. Osteoblasts express several Wnt proteins, which stimulate osteoblastogenesis via a number of different mechanisms, such as attenuating adipocyte differentiation induced by the peroxisome proliferator-activated receptor γ (PPARγ) [16]. Canonical Wnt signaling is transduced through frizzled receptors and low-density lipoprotein receptor-related proteins (LRPs) 5 and 6, which function as co-receptors. Therefore, dysregulation of these receptors is implicated in skeletal diseases. For example, mutations in LRP5 and LRP6 genes conferring gain or loss of function, respectively, lead to high bone mass or osteoporosis [17]. Other regulators of Wnt signaling pathway in bone are antagonist proteins of the Wnt/frizzled receptors and Wnt/LRP complexes, including secreted frizzled-related proteins (sFRPs), Wnt inhibitory factor 1 (WIF-1), sclerostin, and dickkopfs 1 (DKK1). In particular, DKK1 inhibits the canonical Wnt signaling: it binds LRP5/6 causing the internalization and degradation of the two co-receptors [18]. In animal models, overexpression of DKK1 caused significant osteopenia, while lack of DKK1 resulted in increased bone formation. Moreover, DKK1 is capable of altering the ratio RANKL/OPG and therefore regulating the RANK/RANKL/OPG axis. In addition to the mechanisms above mentioned, Wnt signaling pathway also participates in bone metabolism regulation by interacting with bone-derived local factors and systemic hormones, such as PTH and BMPs.
Osteoclasts
Osteoclasts are polarized, multinucleated cells that derive from precursor cells of the monocyte/macrophage lineage, which differentiate into inactive osteoclasts. The bone microenvironment plays an important role in osteoclastogenesis and osteoclast activity, regulating these processes via locally produced cytokines and systemic hormones. RANKL is a potent inducer and a key effector in osteoclastogenesis. It is commonly expressed on the cell surface in osteoblasts and stromal cells, but it is also secreted in a soluble form by activated T cells. Osteotropic factors, such as PTH, 1,25-dihydroxyvitamin D3, and prostaglandins, regulate RANKL production. The interaction of RANKL with its cognate receptor RANK on osteoclasts precursors stimulates osteoclast differentiation by downstream activation of the nuclear factor kappa B (NFk-B) and Jun N-terminal kinase (JNK) signaling pathways. The relevance of the interaction of RANK/RANKL in osteoclastogenesis has been proved also in animal models. Transgenic mice lacking RANK or RANKL were unable to produce osteoclasts and presented with a severe osteopetrotic phenotype [19]. An important protein in balancing RANKL function is its decoy receptor OPG, normally expressed in the bone marrow [9, 20]. Overexpression of OPG leads to severe osteopetrosis in mice, while mice that lack OPG show osteopenia [20]. The ratio RANKL/OPG, therefore, rules osteoclastogenesis.
Osteoclast formation is stimulated by IL-1, IL-6, IL-34, prostaglandins, and macrophage colony-stimulating factor (M-CSF) primarily produced by osteoblasts [21]. Some immune cells, such as T-cells, instead, negatively influence osteoclastogenesis by producing IL-4, IL-8, and interferon γ (IFNγ). Furthermore, active osteoclasts secrete proteases that cause degradation of the mineralized bone matrix leading to release of acids and minerals into the extracellular space. Osteoclasts adhere to the bone surface via αvβ3 integrin, forming an actin ring and secreting acid, collagenases, and proteases that demineralize the bone matrix and degrade matricellular proteins, including type I collagen. It is critical that the osteoclasts adhere to the bone matrix during bone resorption, as the use of inhibitors of osteoclast attachment causes disruption of the bone resorption process [22].
Calcium Homeostasis
Calcium is the primary inorganic component of the mineralized bone matrix. Serum calcium concentration is highly regulated by a complex system of calcitropic hormones, which act at the levels of bone, kidney, and gut. PTH and vitamin D in its biologically active form (calcitriol or 1,25-(OH)2D3) act on these organs and maintain the levels of ionized calcium stable in blood. Serum calcium concentration is maintained within a very narrow range by the interaction of these two calcitropic hormones with their target tissues in bone, kidney, and gut. Under normal conditions, the net calcium exchange from extracellular fluid to these organs is zero [23]. Physiologically, PTH and vitamin D are the most important calcitropic hormones in humans. Calcitonin plays instead a less relevant role. In the bone microenvironment, calcium levels are maintained within a narrow physiologic range (~1.1–1.3 mmol/L) [24]. Active osteoclastic bone resorption causes extracellular calcium (Ca2+) levels to rise up to 8–40 mmol/L [25].
Calcium effects are mediated through the extracellular calcium-sensing receptor (CaSR). CaSR is a G-protein-coupled receptor which responds to high concentration of Ca2+ inhibiting cyclic AMP (cAMP) and activating phospholipase C (PLC) [26]. CaSR is expressed in normal tissues and regulates the secretion of parathyroid hormone-related protein (PTHrP) . In the presence of low concentration of Ca2+, CaSR increases PTHrP secretion, which activates bone resorption and causes release of calcium from the bone matrix. High Ca2+ levels or CaSR agonists reduce PTHrP secretion [27, 28].
Parathyroid Hormone
PTH is an 84 amino acid polypeptide that is secreted by the chief cells of the parathyroid glands. Secretion of PTH is highly regulated by Ca2+ concentration in the extracellular fluid. PTH secretion decreases as Ca2+ concentration increases, in a simple negative-feedback loop [27]. Activation of CaSR leads to the downregulation of PTH at the posttranscriptional level [29]. Another potent inhibitor of PTH secretion is calcitriol, while hyperphosphatemia increases PTH secretion [29–32].
PTH is active in bone, stimulating osteoclastic bone resorption, via osteoblast production of RANKL. In the kidney, instead, PTH stimulates calcium reabsorption and inhibits phosphate reabsorption from renal tubules, and it stimulates renal 1α-hydroxylase, resulting in calcitriol production, which, in turn, increases intestinal absorption of calcium and phosphate. Therefore, PTH biological actions result in increased serum calcium and increased urinary phosphate excretion. PTH signaling is mediated by its receptor, a G-protein-coupled receptor [33]. Following PTH binding, the receptor activates adenylate cyclase, which leads to production of cAMP and activation of protein kinase A (PKA). Although this seems to be the dominant pathway, PTH signal transduction also travels through the PLC/protein kinase C (PKC) route [34, 35].
1,25-(OH)2D3
One of the other important hormones participating in calcium homeostasis is 1,25-(OH)2D3 or calcitriol. 1,25-(OH)2D3 is a biologically active metabolite of the vitamin D sterol family. Vitamin D precursor can be synthesized from 7-dehydrocholesterol inside the skin via exposure to sunlight, or it can be introduced by diet. In the liver, the precursor undergoes hydroxylation at the C-25 position and it is successively hydroxylated at the C-1 position in the kidney , forming 1,25-(OH)2D3 [36–38]. The most important control point of vitamin D metabolism is the renal 1α-hydroxylation of 25-(OH)D3, regulated by phosphate, PTH, and calcitriol concentrations. Low serum phosphate level and PTH increase 1,25-(OH)2D3 production in an independent fashion [39, 40]. Increased levels of calcitriol, instead, downregulate calcitriol production via an autocrine negative-feedback loop that signals through vitamin D receptors in cells of the proximal convoluted tubule in the kidney [41]. The placenta and the granulomatous tissue are other known important sites of calcitriol production [42–44].
1,25-(OH)2D3 increases calcium and phosphate absorption in the gastrointestinal tract, increasing plasma concentration of calcium and phosphate. Moreover, it stimulates PTH ability to promote calcium resorption in the renal tubules, and increases bone resorption [45]. 1,25-(OH)2D3 functions as a differentiation agent for committed osteoclast precursors, which become mature multinucleated cells capable of bone resorption [46, 47]. In all, 1,25-(OH)2D3 function is to guarantee a sufficient amount of calcium and phosphate available for bone matrix mineralization at the bone surface.
Calcitonin
Calcitonin has an uncertain biological role in calcium homeostasis. It directly inhibits osteoclast bone resorption, with a rapid effect within minutes of administration [48]. Calcitonin causes production of cAMP and increase of cytosolic calcium in osteoclasts, resulting in the contraction of the cellular membrane [49–51]. These effects are transient and probably do not play a significant role in chronic calcium homeostasis.
Hypercalcemia of Malignancy
Hypercalcemia is defined as total serum calcium adjusted for protein concentration above 10.2 mg/dl. The most common causes of hypercalcemia are primary hyperparathyroidism and malignancy, and the most common clinical manifestations are neuromuscular, gastrointestinal, and renal symptoms [52]. Hypercalcemia of malignancy is one of the most common paraneoplastic syndromes, with lung, breast, and hematologic tumors being the most frequently associated malignancies [53]. Tumors can secrete humoral factors that unbalance calcium homeostasis acting on bone, kidney, and intestine, and/or local factors in bone, in the case of metastases or hematological tumors, which directly stimulate bone resorption by osteoclasts [52].
Humoral hypercalcemia of malignancy is mostly due to increased PTHrP levels. PTHrP is expressed in many tumors and in normal tissue [54–57]. In addition to mediating hypercalcemia, PTHrP also plays a role in the development and progression of osteolytic bone metastases and tumor cell growth and survival [58–61]. In hematological tumors 1,25-(OH)2D3 mediates hypercalcemia together with PTHrP [62–65]. Increased production of PTH is instead only rarely associated with hypercalcemia of malignancy [52]. Other tumor-secreted humoral factors that contribute to the development of hypercalcemia are IL1, IL6, TGFα, TNF, and granulocyte colony-stimulating factor (G-CSF) [66–73].
Bone Metastases
Certain types of cancer, such as breast, prostate, and lung, have a higher propensity to metastasize to bone. Bone is the third most frequent site of tumor metastasis after the liver and the lung and almost all the patients with advanced breast or prostate cancer present with bone metastases. The spread and metastasis of tumor cells to the skeleton is a complex multistep process highly dependent on the properties and characteristics of tumor cells and bone microenvironment. A tumor cell needs to successfully complete each step of this process in order to establish a secondary tumor in bone. The metastatic process follows sequential events: detachment of cancer cells from the primary tumor, invasion of the adjacent tissues, entry into the circulatory system via the neo-vasculature of the tumor, survival of host immune response and physical forces in the circulation, arrest in the distant capillary bed, and extravasation and growth into the bone [74].
The “Seed and Soil” Model and the Pre-metastatic Niche
A first concept, proposed by Batson in 1940, hypothesized that the vertebral system of veins acts like a conduit for cancer cell dissemination to the skeletal system [75]. However, this hypothesis does not explain the preferential homing of cancer cells to the bone or other sites of metastases. The exact mechanism that drives certain cancer cells to the bone is still unclear, but there is evidence that the bone microenvironment plays an important role in this process. In 1989, Paget proposed the “seed and soil” hypothesis to explain the tropism of tumor cells for specific organs to form metastases. “When a plant goes to seed, its seeds are carried in all directions; but they can only grow if they fall on congenial soil” [76]. In this metaphor, the tumor cells are the seeds that will grow and form metastases only in the microenvironment of the organ that provides a fertile nourishing soil. This concept remains a basic principle of the understanding of tumor metastasis and is a basic underpinning of research in the field today [77]. Moreover, in the case of the bone tissue, destruction of the mineralized matrix is necessary in order for the tumor cells to invade the bone. This bone resorption is mediated by osteoclasts activated by the cross talk between the tumor cells and the bone microenvironment [2].
More recently, the model of the pre-metastatic niche has been formulated. This model proposes that a primary tumor is capable to prepare a conducive microenvironment at a distant site before the disseminated tumor cells arrive at the site and establish metastases [78]. The concept of pre-metastatic niche, hence, involves the action of the primary tumor on the destination site of metastasis through production of tumor-derived growth factors, such as TGFβ, vascular endothelial growth factor A (VEGFA), and placental growth factor (PGF). In response to these factors, hematopoietic progenitor cells (HPCs), macrophages, and other tumor-associated immune cells gather at the metastatic site and prime the “soil” for the arrival of the tumor cells, helping adhesion and invasion [2, 79]. Recent data show that, in preclinical models of melanoma and lung cancer, bone marrow-derived hematopoietic cells expressing vascular endothelial growth factor receptor 1 (VEGFR1) home to the future metastatic site to form cellular clusters that increase fibronectin production in tumor target sites previous to the arrival of the tumor cells [80]. Further evidence of the existence of a pre-metastatic niche is the production of inflammatory chemo-attractants in pulmonary sites in a model of lung metastasis [81]. Although the molecular factors mediating the initial engraftment are still to be completely explained, it seems that the accumulation of myeloid cells, fibronectin, growth factors, and matrix remodeling proteins accelerate the micrometastatic process. Recently, in a model of breast cancer bone metastases, myeloid-derived suppressor cells (MDSCs) isolated from the tumor microenvironment have been shown to differentiate into osteoclasts [82]. Moreover, the recruitment of endothelial progenitor cells contributes to the switch from micrometastatic to macrometastatic phenotype. However, a lack of effects of bone marrow-derived circulating endothelial precursor cells on tumor growth has been reported [83]. The primary tumor determines the site of metastases also through the production of stroma-derived factors [84]. Zhang et al. showed that cancer-associated fibroblasts (CAF) in triple-negative breast carcinoma select cell clones within the primary tumor that thrive on CAF-derived factors CXCL12 and IGF-1. These clones showed high Src activity, which is related to increased Akt/PI3K signaling and bone relapse, and are primed for metastasis in the bone marrow microenvironment, rich of CXCL12 [85].
In the bone microenvironment, the primary tumor conditioning may take place through endocrine-like actions, such as the production of circulating factors that target bone marrow cells and cells in the bone microenvironment, rendering it conducive to tumor colonization. It has been shown that breast cancer cells produce heparanase to increase bone resorption [86]. Other examples are tumor cells and senescent fibroblasts secreting osteopontin (OPN) to promote bone marrow cell recruitment and tumor formation, and osteoclasts producing matrix metalloproteinases (MMPs) to support prostate cancer bone metastasis formation [87–90]. Also, several tumors produce PTHrP , which can promote bone resorption and increase the production of local factors, such as chemokine (C-C motif) ligand 2 (CCL2), in the bone marrow [91–93]. In general, the bone microenvironment is a very fertile “soil” for metastatic cancer cell growth and proliferation because of the abundance of immobilized growth factors, such as TGFβ, IGF1, FGF, PDGF, and BMPs, cytokines, chemokines, calcium ions, and cell adhesion molecules [7, 9]. Many of these molecules are released mostly as a consequence of osteoclastic bone resorption, but also can be produced by stromal and immune cells in the bone marrow. In addition to molecular factors, also physical characteristics of the bone microenvironment, such as hypoxia, acidic pH, and high extracellular calcium concentration, facilitate tumor growth. Unlike in normal calcium homeostasis, in prostate and breast cancer cells PTHrP synthesis is increased by high levels of extracellular calcium. In turn, PTHrP stimulates bone resorption, which increases extracellular calcium concentration, creating a feedback loop [94–97]. Moreover, the bone marrow stromal cells might cross talk and collaborate with the tumor cells in the homing, differentiation, and proliferation processes, through the production of vascular cellular adhesion molecule 1 (VCAM1), cadherin 11, and fibronectin [98]. When tumor cells establish themselves in the bone microenvironment they start producing cytokines and growth factors that stimulate osteoclastic bone resorption both directly and indirectly. This creates a symbiotic relationship between tumor and bone microenvironment alimented by a molecular cross talk that sustains a feed-forward “vicious cycle” of increased bone destruction and tumor growth, leading to the formation of cancer bone metastases.
Bone Colonization
To escape from the primary neoplasm tumor cells first need to adhere to the basement membranes and other surrounding cells through cell adhesion molecules such as E-cadherin and laminin. Then they produce proteolytic enzymes that degrade the basement membrane and the proteins in the extracellular matrix, allowing the tumor cells to invade the surrounding tissues [99]. Among these enzymes are MMPs, a family of zinc-dependent proteinases that degrade extracellular matrix proteins. Clinical evidence indicates that platelets may physically shield tumor cells from the action of the immune system, thus promoting the metastatic process [99].
Tumor cells adhere preferentially to the bone marrow endothelium. Tumor cells homing to bone can use the same physiological mechanism used by hematopoietic stem cells (HSCs) [79, 100–102]. HSCs are attracted and regulated by osteoblasts and bone marrow stromal cells through integrins, such as α4β1, αvβ3 and VCAM1, chemokines, such as chemokine (C-X-C motif) receptor 4 (CXCR4) and chemokine (C-X-C motif) ligand 12 (CXCL12) (also known as stromal cell derived factor (SDF-1)), BMPs, Notch, OPN, and nestin [103–111]. HSC homing is also regulated by bone resorption, as proved in preclinical study. Metastatic prostate cancer cells and probably other cancer cells directly compete with the HSCs for the occupancy of the bone marrow niche [112–114].
Expression of CXCR4 on cancer cells has a major role in tumor cell homing to bone, and its ligand, SDF-1 or CXCL12, is expressed at high levels by osteoblasts and bone marrow stromal cells [100, 115–118]. Several groups have demonstrated a direct role of CXCR4/CXCL12 in breast and prostate cancer cell proliferation, suggesting a role for this pathway in breast and prostate cancer cell homing to bone and establishment of bone metastasis [118–121]. CXCL12 expression by bone marrow stromal cells also upregulates expression of MMP9 and αvβ3 integrin on prostate cancer cells [116–118, 122]. CXCR4 overexpression, along with other bone metastasis signature genes, such as IL11, MMP1, and connective tissue growth factor (CTGF), in breast cancer cell lines increased their ability to metastasize to bone [100].
Integrins expressed on the cancer cell surface interact with proteins of the extracellular matrix that are expressed in the bone microenvironment. VCAM1 is normally expressed by bone marrow stromal cells and constitutes a ligand for α4β1 integrin [123]. Therefore, tumor cells expressing α4β1 integrin are expected to preferentially adhere to bone marrow stromal cells during the metastatic process. In fact, Chinese hamster ovary (CHO) cells transfected with α4β1 integrin were capable to establish bone and lung metastases after intravenous inoculation in nude mice, while non-transfected CHO cells only generated lung metastases [124]. In addition, antibodies against α4β1 integrin or against VCAM1 were able to inhibit the development of bone metastases. Expression on tumor cells of the α2β1, α5β1, and α4β1 integrins, respectively, receptors for collagen I, fibronectin, and VCAM1, has been shown to be involved in the interaction between cancer cells and bone marrow stroma in leukemia, breast cancer, prostate cancer, and myeloma [123–130]. The αvβ3 integrin interacts with fibronectin, vitronectin, OPN, and bone sialoprotein, and its expression in tumor cells is associated with increased bone metastasis, tumor-associated osteolysis, and bone endosteum colonization in breast and prostate cancer [131–134].
CXCR4 activation increases αvβ3 expression on prostate cancer cells and αvβ1 on myeloma cells, suggesting a cross talk between CXCR4 and integrin expression that could promote cancer cell recruitment to bone and bone colonization [116, 117, 135]. Bone-derived growth factors as TGFβ released during bone resorption might also enhance the metastatic potential of cancer cells with a mechanism involving integrin interactions [136].
The bone microenvironment constitutes a very favorable “soil” enriched with numerous factors promoting tumor growth, and the interaction between cancer cells and bone microenvironment can be studied in the different models of metastatic tumor and it represents the basis for cancer bone metastasis development.
Osteolytic and Osteoblastic Metastases
Conventionally bone metastases are classified into two different types: osteolytic bone metastases and osteoblastic bone metastases. However, most bone metastasis patients exhibit both the osteolytic and the osteoblastic component in different degrees, due to the general dysregulation of bone metabolism caused by the tumor affecting bone formation and bone resorption [7].
Osteolytic metastases are more common than the osteoblastic ones. Patients with osteolytic bone metastases suffer severe bone pain, spinal cord compression, pathologic fractures, and hypercalcemia. Breast, lung, renal, and thyroid carcinomas are some examples of cancer that produce osteolytic bone metastases [137]. Different primary tumors may use different mechanisms to activate osteoclasts and cause bone resorption.
Osteoblastic metastases are commonly associated with prostate cancer, but they have been described also in other tumors. Osteoblastic metastases result from increased osteoblast differentiation, proliferation, and activity, leading to excess bone deposition [74]. In patients, osteoblastic metastases lead to bone pain and pathological fractures due to the fragility of the disorganized new bone produced by overstimulated osteoblasts [9].
Osteolytic Metastases: Breast Cancer as the Prototype
The majority of the patients with breast cancer bone metastases present with osteolytic lesions. Breast cancer bone metastases are associated with increased production of factors such as TGFβ, RANKL, PTHrP, IL11, IL8, IL6, and IGF1, which stimulate osteoclastogenesis, tumor growth, and bone resorption [99].
In breast cancer, PTHrP is expressed in 90 % of cases at the bone metastatic site and only in less than 20 % of cases at other metastatic sites [92, 138–140]. These data suggest a crucial role for PTHrP as a determinant for bone metastasis development in breast cancer. Blockade of PTHrP in a mouse model of MDA-MB-231 breast cancer bone metastases decreases size and number of osteolytic lesions and osteoclast number at the bone/tumor interface [92, 141]. MCF-7 is a breast cancer cell line that does not express PTHrP and does not cause bone metastases in vivo. When MCF-7 cells are engineered to overexpress PTHrP, they cause increased osteoclastogenesis and marked bone destruction [142]. PTHrP signals through its receptor, PTHR1, and stimulates RANKL expression by the osteoblasts and inhibits expression of OPG, the decoy receptor for RANKL, by stromal cells, resulting in an increase in osteoclastogenesis and bone resorption [99, 142].
Another important mediator of breast cancer osteolytic bone metastases is TGFβ. TGFβ is released from the mineralized bone matrix in its active form and it increases breast cancer bone metastases via stimulation of PTHrP secretion by tumor cells [6, 143]. TGFβ signaling is mediated through the interaction with the type II receptor, which in turn recruits and phosphorylates the type I receptor [144]. Interference with the expression of these receptors in animal models affects tumor burden and osteolytic lesions [143, 145]. TGFβ stimulates PTHrP secretion mainly via SMAD (mother against decapentaplegic homolog)-dependent signaling pathways, involving SMAD2, 3, and 4, in breast cancer bone metastases. However, PTHrP production can also be enhanced by TGFβ through the p38 mitogen-activated protein kinase (MAPK) signaling pathway [146].
Breast cancer cells commonly express Runt-related transcription factor 2 (RUNX-2) , a transcription factor that regulates osteoblastogenesis. Blockade of RUNX-2 in a mouse model of breast cancer bone metastases reduces tumor burden and decreases bone resorption [147]. Wnt and DKK1 have also been found implicated in osteolytic bone metastases and their role has been extensively investigated [16]. DKK1 expression is higher in patients with breast cancer bone metastases compared to healthy women, breast cancer patients in complete remission, and patients with breast cancer metastases in sites other than bone [148]. Elevated expression of DKK1 is detected in cell lines producing osteolytic or mixed osteoblastic/osteolytic metastases. Conversely, in cell lines associated with osteoblastic metastases, DKK1 expression is not detectable [149].
Several growth factors, such as IL11, IL6, prostaglandin E2 (PGE2), and M-CSF, are produced by breast cancer cells. These growth factors play an important role in osteolytic lesions, inducing osteoclastogenesis and inhibiting osteoblast formation and activity [150, 151]. PGE2 stimulates RANKL expression, resulting in increased osteoclastogenesis [151]. IL11 is commonly expressed in stromal and immune cells, such as epithelial cells and fibroblasts, and its expression is stimulated by both PTHrP and TGFβ [152]. IL11 increased expression is associated with augmented tumor burden, bone resorption, and osteolytic lesions in mouse models of bone metastases [152]. A recent study shows that Jagged1 mediates breast cancer bone metastases by activating the Notch signaling pathway in stromal bone cells, stimulating IL6 release from osteoblasts, and activating osteoclast differentiation. Jagged1 is also a downstream mediator of TGFβ signaling and the use of a γ-secretase inhibitor reduces Jagged1-mediated bone metastases [153].
In the bone microenvironment, osteogenic cells secrete placental growth factor (PlGF) , which expression is enhanced by the presence of metastatic breast tumor cells. Inhibition of host-derived PlGF in a mouse model of breast cancer bone metastases reduces incidence, number, and size of bone metastases. PlGF blockade inhibits osteoclastogenesis by preventing upregulation of RANKL and the autocrine osteoclastogenic activity of PlGF, and reduces the engraftment of tumor cells in the bone microenvironment by inhibiting their interaction with the matrix components [154].
IGFs are also released in the tumor microenvironment during osteolysis and appear involved in breast cancer cell proliferation in bone [155, 156]. IGF-1 and IGF-2 are the most abundant factors in the bone matrix, followed by TGFβ [4]. Neutralizing antibodies against IGF-1 receptor abrogate the ability of bone-resorbing cell-conditioned medium to stimulate breast cancer cell proliferation [3, 156]. This indicates that IGF-1 signaling pathway has a role in osteolytic bone metastasis formation.
Recently, microRNAs have also been identified as mediators of osteolytic bone metastases, and they have been proposed as both prognostic biomarkers and therapeutic targets [157, 158]. Ell et al. identified a microRNA expression signature in differentiating osteoclasts exposed to metastatic tumor cell-conditioned media, partially determined by activation of NFkB signaling by metastatic tumor cell-secreted intracellular adhesion molecule (sICAM1). In vivo, intravenous injection of microRNAs downregulated during osteoclastogenesis, miR-141 and miR-219, reduced bone metastases inhibiting osteoclast activity. Serum levels of microRNAs that are upregulated during osteoclastogenesis, miR-16 and miR-378, and sICAM1 were correlated with bone metastasis burden [157] (Fig. 2.2).


Fig. 2.2
Osteolytic bone metastases
Osteoblastic Metastases: Prostate Cancer as the Prototype
Osteoblastic metastases, unlike osteolytic metastases, are generated by tumor cell production of factors stimulating osteoblastogenesis, osteoblast proliferation, and new bone deposition [74, 159, 160]. Prostate cancer is the prototype of cancer, which shows predilection to form osteoblastic bone metastases. However, osteoclast activity is still an important driver of this process as bisphosphonates reduce skeletal-related events in prostate cancer [161].
One important determinant of prostate cancer osteoblastic metastases is endothelin 1 (ET-1). ET-1 is a potent vasoconstrictor and a potent osteoblast stimulatory factor via endothelin A receptor (ETAR) activation [162, 163]. Use of the ETAR antagonist, atrasentan, prevented osteoblastic lesions and reduced skeletal morbidity in patients with advanced prostate cancer [164]. Atrasentan also decreases osteoclastic bone resorption in patient trials [165]. However, in phase III clinical trials in patients with metastatic castration-resistant prostate cancer, atrasentan alone or in combination with docetaxel failed in delaying disease progression and improve overall survival [166–168]. Downstream of ET-1 signaling pathway there are important factors, such as IL6, Wnt5a, CTGF, and RANKL [99]. ET-1 inhibits DKK1, which works as a suppressor of the Wnt signaling pathway, and DKK1 overexpression in prostate cancer cells reduces Wnt signaling and leads to osteolytic lesions in the bone [169, 170]. However, DKK1 overexpression does not cause reduction of the basal osteoclast activity [169]. Overexpression of Wnt-1 and β-catenin has also been described in patients with advanced prostate cancer. This observation indicates a role for the Wnt signaling pathway in the pathogenesis of prostate cancer bone metastases [171].
Paradoxically, osteoblastic bone metastases nearly always express PTHrP. A partial explanation to this is that the prostate-specific antigen (PSA) , which induces an osteoblastic phenotype in animal models [172], is a serine proteinase able to cleave PTHrP [173, 174]. The cleaved NH2-terminal fragment of PTHrP fails to activate the PTH/PTHrP signaling pathway, but can bind and activate ETAR [91, 175]. In experimental models, PTHrP fragment 1-16 enhances osteoblast proliferation and new bone deposition at similar levels to ET-1. Moreover, other two fragments, 1-20 and 1-23, of PTHrP had a marked bone anabolic effect, which was suppressed by the ETAR antagonist, atrasentan [91]. Evidence suggests that PSA might play a similar role in breast cancer bone metastases. PSA is commonly expressed in breast cancer [176]. IGF cleavage from its binding protein and TGFβ cleavage to its active form operated by PSA might contribute to osteoblastogenesis stimulation in breast cancer [177, 178].
There are other factors that have been found implicated in osteoblastic bone metastasis, such as TGFβ, BMPs, PDGF, FGF, IGF-1, adrenomedullin, and several proteases [99]. The serine protease urokinase (uPA) has been shown to be involved in prostate cancer bone metastasis formation in mouse models [179]. Experimental data suggest a dual role for uPA, where the carboxy-terminal domain might participate in tumor invasiveness and growth factor activation, while the amino-terminal domain might stimulate tumor growth [74]. BMP-7, instead, preserves the epithelial phenotype of prostate cancer cells, preventing the epithelial/mesenchymal transition, and its expression is decreased in more invasive and metastatic cells [180]. Other examples are FGF-1 and FGF-2 produced by prostate cancer cells, which could function as factor stimulating osteoblast proliferation [74]. Moreover, CTGF and adrenomedullin are both osteoblast-stimulating factors and are produced by several tumors [181, 182].
The Vicious Cycle
Bone metastases thrive in bone by promoting a feed-forward vicious cycle involving tumor cells and the bone microenvironment components (osteoblasts, osteoclasts, and bone matrix) [183]. The mineralized bone matrix is a rich source of physical factors, such as hypoxia, acidosis, and calcium, and several growth factors. In osteolytic bone metastases, bone resorption by osteoclasts releases growth factors and calcium from the mineralized bone matrix [4]. These factors act on the tumor cells stimulating proliferation and tumor growth. Stimulated tumor cells produce high levels of PTHrP , further inducing osteoclastogenesis and bone resorption. Growth factors released from the bone matrix, such as TGFβ, type I collagen, osteocalcin, and IGFs, function as chemotactic factors for tumor cells in a integrin-dependent fashion [184, 185]. In osteoblastic bone metastases, tumor cells produce factors that stimulate osteoblast proliferation and differentiation, and new bone deposition, such as ET-1. Osteoblasts, in turn, express and secrete growth factors that enhance tumor growth in bone [52, 159, 160]. Recent studies unveil the implication of several microRNAs expressed by tumor cells and bone microenvironment cells in the vicious cycle, and their role in invasion and homing of cancer to bone [157, 186, 187]. Understanding of the mechanisms responsible for bone metastases will lead to better therapy of established disease and prevention of new disease. Most current bone-targeted therapies inhibit osteoclastic bone resorption (bisphosphonates and RANKL antibody), the main cellular driver of the tumor-bone interactions responsible for the morbidity associated with bone metastases (Fig. 2.4).


Fig. 2.4
The vicious cycle
References
1.
Roudier MP, Morrissey C, True LD, Higano CS, Vessella RL, Ott SM. Histopathological assessment of prostate cancer bone osteoblastic metastases. J Urol. 2008;180(3):1154–60.PubMedCentralPubMed
2.
Weilbaecher KN, Guise TA, McCauley LK. Cancer to bone: a fatal attraction. Nat Rev Cancer. 2011;11(6):411–25.PubMedCentralPubMed
3.
Yoneda T. Mechanisms of preferential metastasis of breast cancer to bone—(Review). Int J Oncol. 1996;9(1):103–9.PubMed
4.
Hauschka PV, Mavrakos AE, Iafrati MD, Doleman SE, Klagsbrun M. Growth factors in bone matrix. Isolation of multiple types by affinity chromatography on heparin-Sepharose. J Biol Chem. 1986;261(27):12665–74.PubMed
6.
Pfeilschifter J, Mundy GR. Modulation of type beta transforming growth factor activity in bone cultures by osteotropic hormones. Proc Natl Acad Sci U S A. 1987;84(7):2024–8.PubMedCentralPubMed
7.
Bussard KM, Gay CV, Mastro AM. The bone microenvironment in metastasis; what is special about bone? Cancer Metastasis Rev. 2008;27(1):41–55.PubMed
8.
Goldring SR, Goldring MB. Eating bone or adding it: the Wnt pathway decides. Nat Med. 2007;13(2):133–4.PubMed
9.
Roodman GD. Mechanisms of bone metastasis. N Engl J Med. 2004;350(16):1655–64.PubMed
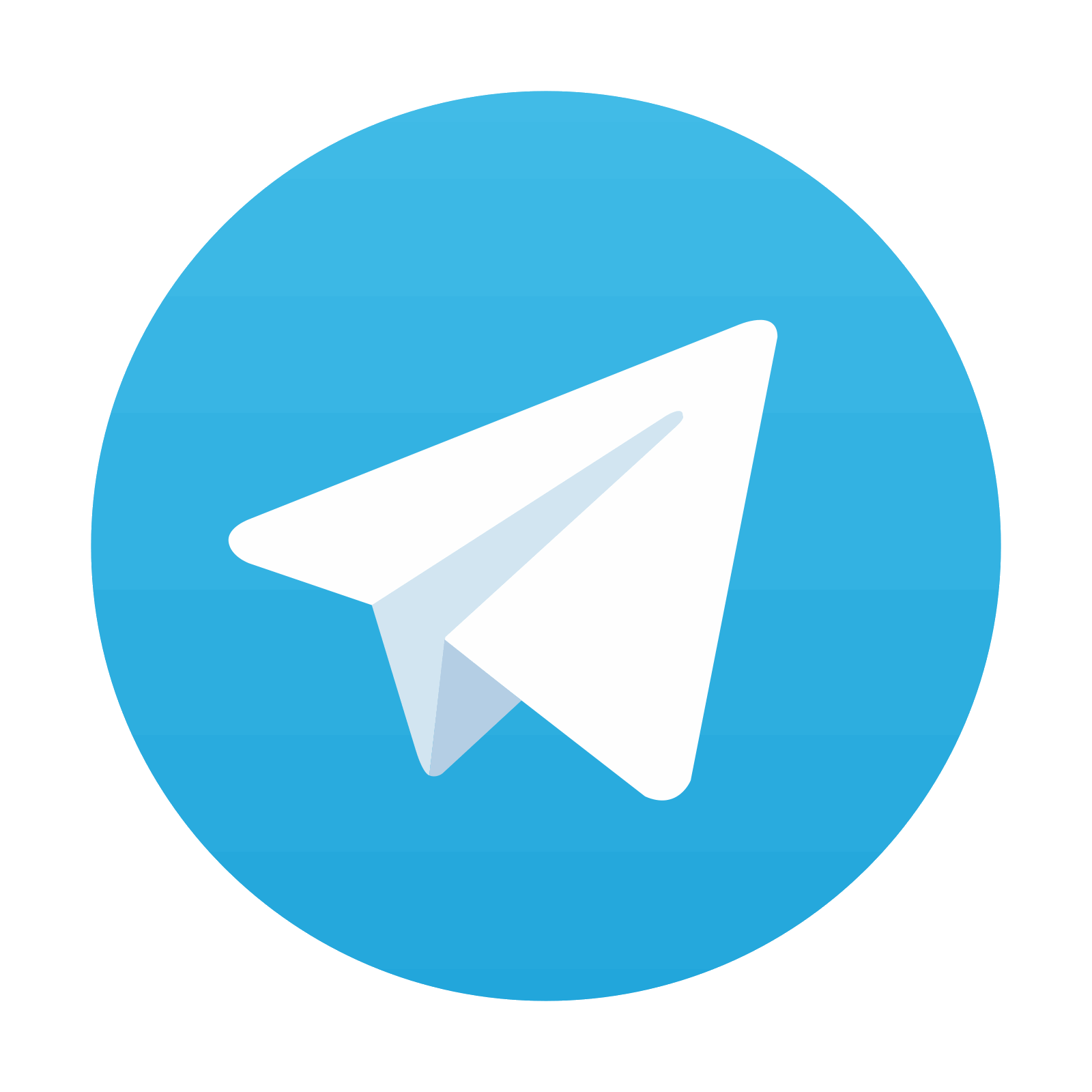
Stay updated, free articles. Join our Telegram channel
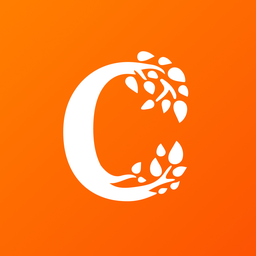
Full access? Get Clinical Tree
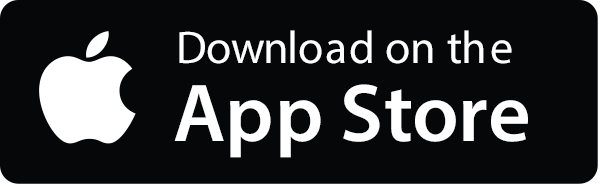
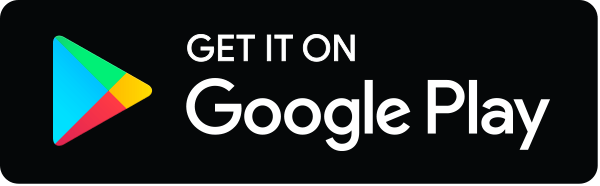