Fig. 1
Official grading sheet for the International Standards of Neurological Classification of Spinal Cord Injury (Source: Courtesy of American Spinal Injury Association, Atlanta, Georgia)
Regionalization and Pathophysiology of SCI-Associated Osteoporosis
Traumatic SCI is one of several neurological disorders leading to disuse osteoporosis and resultant fractures, but it differs from many other clinical conditions in the rate of osteoporosis development. Approximately two weeks after acute spinal cord injury, a rapid process of bone resorption exceeding that of bone formation begins to occur, primarily due to upregulation of the osteoclasts [4, 5]. Given its faster turnover rate [6, 7], trabecular bone is more commonly affected than cortical bone in the first four months following SCI. During the first six months following acute SCI, markers of bone formation range from mildly depressed to slightly elevated, depending on the study cited [5, 8, 9]. Even if markers of formation are slightly increased, this compensatory process is not sufficient to counteract the significant bone resorption that occurs concurrently. Roberts and colleagues [9] observed a rapid rise in urinary values of total and free deoxypyridinoline, total pyridinoline, and NTX in the immediate period following injury. Elevations in bone resorption markers began at one week post injury, peaked at 16–20 weeks, and then gradually declined. At their highest value, some markers measured ten times the upper limit of normal. Given that bone loss begins shortly after injury, with the bulk of loss in BMD occurring within 4–6 months, aggressive steps should be undertaken to prevent bone loss shortly after injury [10–12].
Radiographic evidence suggests that an estimated 25 % of BMD below the level of injury is lost within the first four months following acute SCI, progressing to a 33 % loss by 16 months post injury [1, 13] and leaving patients at or near the fracture threshold [1]. Additional investigations extending the time from injury to two years estimate a BMD reduction of 30–40 % at the femoral neck, 37–52 % at the distal femur [14, 15], and 50–70 % at the proximal tibia [8, 15], with the majority of this loss occurring during the first 12 months. Bone loss subsequent to SCI occurs specifically below the level of injury. In some regions, it progresses at 1 % per week in the acute phases post injury [16]. Relative to other conditions including space travel, where losses are 0.25 % per week [17], and bed rest in otherwise neuro-intact patients, where bone loss is 0.1 % per week [18], bone loss due to acute SCI is 10–40 times greater and to date, appears to be more resistant to treatment. Intervention is needed early after injury to halt the rapid progression of bone loss, but such measures are difficult to implement given the complex metabolic factors leading to accelerated bone loss as well as medical comorbidities that may exist during the acute care phase of hospitalization.
Specifically, individuals with SCI develop sublesional osteoporosis, namely, bone loss below the level of paralysis [14]. Bone abnormalities after SCI develop at an increased rate in the following areas: proximal femur, distal femur, and proximal tibia [8, 9]. In contrast, persons without SCI exhibit higher rates of osteoporotic fractures in the axial skeleton. Areas of the appendicular skeleton in the SCI population are highly susceptible to bone demineralization due to decreased weight-bearing affecting these areas when patients are in lying and sitting positions [14].
The regional BMD in the area below the injury, rather than the overall BMD, is among the strongest predictors of future “fragility fractures” or ones that occur under conditions of minimal or low impact in the absence of trauma [19, 20]. The most significant risk factor for osteoporosis after SCI is the completeness of injury by American Spinal Cord Injury Association (ASIA) grades A through E [21], with patients who have complete injuries at greater risk than those with motor preservation below the injury level. Those with ASIA impairment A (complete) injuries as shown in Fig. 2 are more likely to experience sublesional bone loss than those with motor incomplete injuries (ASIA impairment C or D) who have retained some ability to move their lower extremities. However, having an incomplete SCI (Fig. 3) does not imply the ability to walk or even bear weight on the lower extremities. Originally developed by ASIA, this grading scheme has been adopted as the International Standards for Neurological Classification of Spinal Cord Injury. A higher incidence of sublesional osteoporosis is also observed with increasing number of years post injury [6, 22], increasing age [21, 23], female gender [24] and onset of SCI prior to age 16, when peak bone mass has not yet been achieved [14].



Fig. 2
MRI of a complete cervical SCI. MRI in sagittal view of a 30-year-old male with traumatic C6 ASIA impairment A spinal cord injury due to a motor cycle accident. The image shows a C6 burst fracture with retropulsion of fragments resulting in spinal cord contusion and narrowing of central canal (Source: Courtesy of Thomas Jefferson University, Department of Radiology)

Fig. 3
MRI of an incomplete cervical SCI, shown in sagittal view of an 81-year-old male with a traumatic C4 ASIA impairment C spinal cord injury, due to a fall. There are multi-level degenerative changes in the cervical spine most noticeable at the C4–5, where abnormal cord signal is also seen. In addition, increased T2 signal is seen within the disk space at C5–6, contiguous with prevertebral fluid (Source: Courtesy of Thomas Jefferson University, Department of Radiology)
Epidemiology of Osteoporosis Following SCI
SCI patients with more severe injuries (complete or near-complete) are at heightened risk of developing osteoporosis, particularly if an individual is older and has a cervical level of injury, minimal spasticity, and a longer chronicity of injury [8]. The percentage of patients affected with osteoporosis is also dependent on the years since injury, gender, and neurological level of injury (cervical, thoracic or lumbar, complete or incomplete). Although bone loss inevitably occurs in the vast majority of individuals with motor complete as well as substantial numbers of motor incomplete patients with SCI, additional factors contribute to the rate and extent of bone loss below the level of injury. Apart from the severity of SCI and neurological level of injury, nutrition, lifestyle/activity levels, smoking, and family history must also be taken into account. However, these factors may be more relevant in contributing to pre-injury BMD than in preventing bone loss after SCI. The greater the deficiency in an individual’s bone density at the time of injury, the more bone a patient could theoretically lose before becoming osteoporotic.
Gifre and colleagues have developed a prediction model for development of osteoporosis 12 months after injury [25]. Their examination of 35 patients with a diagnosis of motor complete SCI of six months or less revealed that total femur BMD <1 g/cm2 (RR 3.61) and lumbar BMD <1.2 g/cm2 (RR 2.83) were the strongest predictive factors for development of osteoporosis by one year post injury. Both factors together suggested a probability of 96.8 % that a given individual would develop osteoporosis. Although the authors based this model on a relatively small sample, it included primarily young males with a mean age of 30, likely healthier than some populations of patients, with many still young enough not to have reached peak BMD. Overall, 52 % of their sample developed osteoporosis by one year. Other studies suggest a wide range of percentages by the first year. By patient self-report covering over 1,000 Canadians (approximately 55 % with complete injuries), 21 % stated that they had received a diagnosis of osteoporosis [26]. Given the self-report study design, this estimate is likely to be low; the results were further influenced by the inability to define the timeline of development of osteoporosis from the onset of SCI. In other investigations, prevalence of osteoporosis in SCI patients was found to be much higher; many subjects who do not reach threshold by DXA for −2.5 standard deviations below young normal by a T-score are categorized as osteopenic (T-score of −1.5 to −2.49).
In a survey by Hammond et al. involving 115 female and 264 male chronic SCI patients seen in an outpatient clinic, 34.9 % met the clinical definition of osteoporosis, while another 46.7 % were classified as osteopenic [27]. Lazo and colleagues reported a high incidence of osteoporosis in a sample of male veterans at an average of 15 years post SCI (median age of 55). In their trial, 61 % met the definition of osteoporosis, while another 19.5 % were osteopenic [28]. Perhaps the highest percentage of osteoporosis was evident in a study by Shojaei et al. of SCI veterans in Iran. In this investigation, 81.5 % demonstrated osteoporosis in the femoral neck, and another 13.1 % were found to have osteopenia. As in similar studies, the lumbar spine had lower degrees of osteoporosis due to loading in the lower back during transfers. In the lumbar lateral spine, a view which is more sensitive than the anterior–posterior view (as described in earlier chapters of this book), 16.7 % were osteoporotic while 18.2 % were osteopenic [29]. Table 1 summarizes the many investigations that have shown sublesional osteoporosis in SCI, with a mix of prospective and cross-sectional studies [15, 22, 24, 30–36].
Table 1
Sublesional bone mineral density in spinal cord-injured patients (arranged by prospective/cross-sectional studies)
Author | Type of study | Duration after injury | Male/female | Age (years) | Skeletal site measured | BMD (Z-score, SD, or % loss or reduction of BMD) |
---|---|---|---|---|---|---|
Biering et al. [30] | Prospective | Nine days–53 months | 8:0 | Femoral neck | −30 to 40 % | |
Distal femur | −48 % | |||||
Proximal tibia | −45 % | |||||
Femur diaphysis | −25 % | |||||
Tibia diaphysis | −25 % | |||||
Garland et al. [31] | Prospective | 6:0 | Distal femur | −27 % | ||
Proximal tibia | −32 % | |||||
Uebelhart et al. [32] | Prospective | > Six months | 6:0 | Lower extremity | −6.4 % | |
Warden et al. [33] | Prospective | 1–6 months | 15:0 | 19–40 | Calcaneus | −7.5 ± 3.0 % |
Proximal Tibia | −5.3 ± 4.2 % | |||||
Dauty et al. [15] | Cross-sectional | >One year | 31:0 | 18–60 | Femoral neck | −30 % |
Femoral trochanter | −39 % | |||||
Distal femur | −70 % | |||||
Proximal tibia | −52 % | |||||
Finsen et al. [34] | Cross-sectional | Seven months–33 years | 19:0 | 15–64 | Tibia distal diaphysis | −26 % |
Tibia distal metaphysic | −45 % | |||||
Zehnder et al. [22] | Cross-sectional | < One year | 16:0 | 18–60 | Femoral neck | −0.03 ± 0.25 SD |
< One year | 16:0 | Tibia epiphysis | −0.34 ± 0.22 SD | |||
1–9 years | 38:0 | Femoral neck | −1.65 ± 0.17 SD | |||
1–9 years | 38:0 | Tibia epiphysis | −3.81 ± 0.13 SD | |||
10–19 years | 31:0 | Femoral neck | −1.76 ± 0.25 SD | |||
10–19 years | 31:0 | Tibia epiphysis | −4.00 ± 0.21 SD | |||
20–29 years | 13:0 | Femoral neck | −1.76 ± 0.28 SD | |||
20–29 years | 13:0 | Tibia epiphysis | −4.12 ± 0.24 SD | |||
Garland et al. [24] | Cross-sectional | 2–8 years | 0:6 | 20–30 | Knee | −37.90 % |
Hip | −17.50 % | |||||
3–30 years | 0:16 | 31–50 | Knee | −41.30 % | ||
Hip | −25 % | |||||
9–44 years | 0:9 | 53–77 | Knee | −47 % | ||
Hip | −25.50 % | |||||
Jones et al. [35] | Cross-sectional | 7–372 months | 20:0 | 17–52 | Femur | −27 % |
Hip | −37 % |
The Role of Vitamin D in SCI
In addition to the nutritional prevention strategies outlined earlier in this book, spinal cord injury patients have some specific risk factors for malnutrition. Low levels of serum 25-hydroxy vitamin D occur in substantial numbers of persons with acute and chronic SCI [37–41]. In humans, sunlight is the primary source of vitamin D and typically provides up to 90 % of the daily requirement [42, 43]. The capacity of a spinal cord-injured person to absorb vitamin D from the sun is limited by seasonal and related extremes of heat, cold, humidity, or dryness; skin pigmentation; functional mobility; and availability of assistance from caregivers. Individuals with tetraplegia frequently depend on others to get them out of bed, but such assistance may not be available at desired times of peak sunlight. In addition, those with injures above T6 face thermoregulatory challenges and are highly sensitive to extreme heat and humidity, while others with respiratory issues are adversely affected by very dry climates.
All persons with insensate skin are at risk of developing sunburn and those with complete spinal cord injuries are at particular risk. Although use of sunscreen with SPF >8 will block harmful ultraviolet rays and prevent such burns, cutaneous production of vitamin D3 is reduced by more than 95 % [42–44]. In most climates of the United States, exposure to sunlight for only 15 minutes between 10 am and 3 pm in spring, summer, and early fall is sufficient to obtain adequate vitamin D in persons with fair skin. Persons living in far northern states would incur this benefit only later in the spring or in early fall [45]. Although such limited time will not result in sunburn, some with cervical or upper thoracic neurologic levels of SCI cannot tolerate even this short period, due to temperature dysregulation related to alterations in the autonomic nervous system.
Research examining levels of vitamin D in SCI patients according to season and ethnicity has demonstrated that only 35 % of acute SCI patients injured in summer months and 16 % in winter months actually have therapeutic levels of vitamin D (>32 ng/ml). The remainder required supplementation to achieve therapeutic levels. Findings are summarized in Table 2 [39].
Table 2
Vitamin D levels in acute traumatic SCI patients according to season
Vitamin D levels in summer and winter in acute traumatic SCI patients | ||
---|---|---|
Serum 25-OH vitamin D (ng/ml) | Summer (% total/season) | Winter (% total/season) |
Therapeutic (≥32 ng/ml) | 34.5 | 15.4 |
Subtherapeutic (20–31.99) | 31.0 | 30.8 |
Insufficient (13–19.99) | 27.6 | 23.0 |
Deficient (0–12.99) | 6.9 | 30.8 |
Epidemiology of Insufficiency Fractures Subsequent to SCI
Frequency and Location of Fractures
Patients with complete as opposed to incomplete SCI are at exceptionally high risk of developing fractures in the years subsequent to injury, with the most common sites being the distal femur and proximal tibia [16]. While Bauman et al. focused on hip fractures caused by falls to the ground from wheelchairs or during wheelchair sports, the majority of fractures occur in the lower extremity in the absence of, or with minimal trauma, often as a result of transferring to a car from a wheelchair; a low velocity fall on an outstretched knee; bumping into unforeseen objects [26]; or the sudden stopping of a moving vehicle, prompting rapid forward flexion of the femur, even while the patient remains belted in a sitting position. In a study by Akhigbe and colleagues, the four fractures attributable to motor vehicle accidents occurred in the femur. Similar to other studies, most fractures arose from wheelchair-related activities (43 %) which included transfers, although transfers not involving the chair also accounted for 22 % of fractures [46].
The likelihood of fracture occurrence without antecedent trauma is closely related to the level of BMD in the area at risk (sublesional). According to Garland, the threshold for increased fracture risk is achieved when BMD has declined by 36 % [47]. However, this percentage cannot be taken as an absolute measure of fracture risk, since the initial BMD of a given patient is of paramount importance [25].
While there has been more extensive research on osteoporosis following SCI, fewer studies on fracture incidence have been published. Many investigations involve not only a wide range of years since SCI but also heterogeneous ages and levels of injury. Pelletier et al. [26] studied a 1-year fracture incidence in patients with SCI, with both baseline status and the follow-up findings obtained by phone/website questionnaire. Among the entire cohort of 1,137 patients, 84 or 7.8 % experienced a fracture over the study period. Only 55 % of the subjects had a complete SCI. Factors strongly associated with increased fracture risk were motor and sensory complete SCI (OR = 2.2) and motor complete but sensory incomplete SCI with OR of 1.7, and the presence of three or more of the following risk factors: neurological level of injury, age at injury, duration of injury, or female gender. Since this was only a 1-year evaluation, fracture incidence was lower than other studies. Gifre et al. examined incidence of fracture in the initial 10 years following SCI and found that 25 % of those with complete injuries had experienced at least one fracture since leaving the inpatient rehabilitation unit, with most occurring 6–10 years after injury—a finding that was four times the rate of fracture among the incomplete patients in their study.
In the study above by Akhigbe and colleagues [46], fracture incidence in a group of veterans studied for five years was 13.7 % in the years of evaluation, but over one-third had a fracture that occurred following their SCI but before initiation of the study observation period. In an examination of 3,125 patients over a 6-month interval, Morse et al. reported that approximately 10 % had experienced low-impact fractures below the level of their SCI (study excluded high impact fractures) [20]. Notable findings from this investigation were a longer than normal length of hospitalization (35 days) due to the complications linked to the fracture including nonunion/delayed healing, increased muscle spasms as well as pain (in incomplete SCI patients), autonomic dysreflexia, and heterotopic ossification at the fracture site. The authors further stated that no individual with a fracture-related admission underwent an evaluation for osteoporosis during that hospital stay. In 83 % of patients studied by Akhigbe et al., the presence of a new fracture prompted the need for modified or costly new adaptive equipment in the form of wheelchairs, transfer equipment, or lower limb braces.
Regrettably, the majority of patients examined by Pelletier et al. [26] and Gifre et al. (the 2016 study) failed to receive proper diagnostic workup in the form of a DXA scan subsequent to the fracture. Also, both investigations indicate a sharp discordance between those individuals diagnosed with osteoporosis along with those diagnosed with an SCI-related osteoporotic fracture and the actual number of patients who were referred for and received treatment. For example, the study by Morse et al. [20] indicated that in the year prior to the low-impact fracture, 13 % of patients were taking calcium supplements, 33 % took vitamin D, and 2 % were prescribed bisphosphonates, with no patients on teriparatide. In the first year after the fracture, no substantial changes to prescription medications for osteoporosis were seen. Calcium intake increased to 30 %; vitamin D intake went from 33 % to 38 %; bisphosphonate prescriptions increased from 2 % to 7 %; and no patients were prescribed teriparatide. The above data suggests large numbers of the study sample remained untreated.
Fractures and Their Effects on Quality of Life and Mortality
In the investigation by Pelletier [26], those who experienced a fracture commented on how their overall functioning and activities of daily living have been affected. While 22.6 % reported the fracture affected them “not at all” or “very little,” 21.4 % admitted the their life was affected to some extent, while a concerning 56 % declared that fracture affected their life either “completely” or “to a great extent.” Other findings indicated that medical complications including increased pain in those with residual feeling, heighted lower extremity spasticity, and muscle contractures causing obliquity and risk of pressure ulcers impacted functional mobility and participation in activities of daily living. Independence is compromised by the above factors. Moreover, if pressure ulcers develop, prolonged bed rest may be needed, leading to further medical complications such as deep vein thrombosis, orthostatic hypotension, and potentially depression [20, 25, 26, 46, 48].
In addition to quality of life, fractures can alter life expectancy. Carbone et al. [48] investigated the mortality associated with lower extremity fracture and determined that the hazard ratio (HR) for all SCI participants was 1.38 (complete and incomplete patients) and 1.46 for SCI veterans of all ages with exclusively complete SCI. However, for older men (≥age 50) with complete SCI, the hazard ratio is significantly higher at 3.13. In contrast, younger men (<age 50) with complete SCI had a comparatively lower HR of 1.71. The above reports indicate that not only the quality of life but also its duration can be impacted by fractures subsequent to SCI-related osteoporosis.
Management of Osteoporotic Fractures
In patients with SCI, the primary goal is fracture prevention. Among those with chronic SCI, 25–49 % ultimately experience a fragility fracture [49, 50]. For patients who are unlikely to ambulate, conservative treatment with a soft splint has given way to more aggressive intervention. Untreated fractures in all sites below the level of spinal cord injury can be complicated by increased spasticity, autonomic dysreflexia, and limb deformity after healing that make transfers more difficult not just during the fracture recovery period but long afterward [51]. Femoral neck fractures heal poorly and are among the most challenging to treat. Healing is complicated by bone instability and migration of the pelvis proximally, causing pelvic obliquity, altered sitting balance, and ultimately ischial pressure ulcer development. Plaster casts have been shown to cause skin breakdown unknown to the patient or vascular compromise [50]. Since pinning often results in nonunion, endoprosthetic replacement is recommended but can be compromised by subluxation for adductor spasticity. If prosthetic replacement seems most beneficial for the patient, the physiatrist may wish to consider inpatient rehabilitation admission after the procedure, specifically to provide spasticity management along with prevention of autonomic dysreflexia. Pressure mapping to prevent pressure ulcers and review of safety with functional mobility, particularly transfers, would be additional goals of a rehabilitation stay.
The proximal tibia and distal femur are the locations with the greatest degree of bone loss from immobilization following spinal cord injury. In a series of nine patients with spinal cord disorders of mixed etiologies, each with at least one lower extremity long bone fracture, Sugi et al. [52] demonstrated superior patient outcomes relative to earlier studies. All nine subjects returned to their prior functional baseline at their final post-op outpatient visit. No participants experienced a perioperative or postoperative complication, including nonunion or infection. Significant improvement in life satisfaction was observed postoperatively, using the spinal cord injury quality of life measure. With the combined effects of an increased life expectancy after SCI and greater fracture incidence in persons over age 55, the cost physically and emotionally to the patient and financially to the patient and healthcare system will rise in the coming years [10–12, 53, 54]. Given the increasing availability of robotic ambulation devices and assisted-walking technologies involving full-body bracing, patients with complete paraplegia may be candidates for a walking program. Participation will be possible only for those subjects with reasonable bone density who have no contraindications of active unstable fractures.
Treatment
Nonpharmacologic Interventions
A number of nonpharmacologic interventions have been explored to prevent and treat bone loss secondary to SCI. Effects of gravity, mechanical and electrical stimulation, and other forms of exercise or positioning are utilized in an effort to improve BMD. Multiple studies have found no significant change in bone density post static weight-bearing (standing frame) [55, 56] or partial body weight-supported treadmill training [57, 58]. Researchers have produced mixed results in their investigations of functional electrical stimulation (FES) [32, 59, 60]. Recent years have shown more promise with the latter modality, but the onset of therapy, in relation to the time from acute SCI, the age and health of the population tested, and the duration of weeks of treatment, has varied, contributing to inconclusive results when a number of studies are taken into account. Table 3 summarizes the results of a number of investigations using FES in patients with acute SCI [61–65].
Table 3
Studies using FES/EMS to improve BMD in acute SCI patients
Source | Modality studied | Number and demographics of patients studied | Outcomes/findings |
---|---|---|---|
Arija-Blazquez et al. [61] | Electromyostimulation (EMS) for 47 minutes/day, five days/week | n = 8, male patients with acute SCI, ASIA A, eight weeks post injury. Control versus EMS | EMS produced no significant difference in bone biomarkers or BMD changes compared to control |
Eser et al. [62] | FES cycling | n = 38, para- and tetraplegic patients 4–5 weeks post injury | Both control and FES showed reduced tibial cortical BMD within 3–10 months. FES did not significantly attenuate bone loss |
Ergometry for 30 minutes, three days/week for duration of rehabilitation (mean six months) | |||
Clark et al. [63] | Discontinuous FES to lower limb muscles 15 minutes, 2× day, five days/week over five months | n = 23, SCI (C4-T12) patients (ASIA A-D) FES versus control | FES and control differed significantly at three months but not thereafter |
Lai et al. [64] | FES cycling | n = 24 SCI (C5-T8) patients 26–52 days post injury (control vs. FES). Mean age 28 years | BMD decrease rate in distal femur was significantly less in FES group than the control group during first three months. No significant difference after three months |
Ergometry for up to 30 minutes, three days/week, three subsequent months | |||
Sheilds et al. [65] | NMES on one leg, other leg as control. Exercise for ≥ two years | n = 7, ASIA A, above T12. SCI injury within six weeks | 31 % higher trabecular BMD in trained limbs versus untrained limbs |
Pharmacologic Treatment
Initial interventions often involve supplemental calcium and vitamin D, but these measures are insufficient to prevent fractures or enhance bone density in the absence of additional forms of treatment. Multiple investigations have demonstrated that avoidance of calcium in the acute phase following acute SCI is not a risk factor for the development of kidney disease; in fact, in most cases, eliminating or restricting dietary calcium will only predispose patients to additional early bone loss [16].
Thiazide Diuretics
Thiazide diuretics may be a new avenue of prevention of osteoporosis through their mechanism of preventing urinary calcium excretion, therefore promoting increased BMD. A meta-analysis of 21 observational studies, comprising over 400,000 patients with SCI of all severities who were prescribed thiazide diuretics for other medical conditions, showed a 24 % reduction in risk of hip fractures [66]. Carbone et al. [48] examined thiazide use in a 5-year cohort study of 6,969 veterans with varying levels of SCI. Of the 1,433 who used thiazides (HR = 0,750) for medical purposes other than bone preservation, a one-quarter risk reduction was observed, particularly in patients who were also prescribed vitamin D supplements.
Oral Bisphosphonates
Several groups have explored the use of various bisphosphonates for prevention of bone loss in acute and chronic SCI. Zehnder et al. focused on the use of oral alendronate plus calcium and demonstrated that this combination resulted in maintenance of pretreatment BMD, while the group receiving calcium alone had a steady decline in BMD [67]. Another trial found that subjects receiving weekly alendronate administration experience a less notable decline in BMD than those given a placebo [68]. However, giving oral alendronate in the setting immediately following SCI poses a number of challenges. Limitations to achieving an upright posture include postoperative spinal stability precautions, delay in brace fitting needed to maintain stability in sitting position, autonomic effects of orthostasis, and pain. Erosive esophagitis may occur following consumption of oral bisphosphonates, unless patients are able to sit fully upright after administration of medications [67, 68]. Because IV formulations can be administered in the supine position, investigators have explored the use of IV bisphosphonates in the setting of acute SCI.
Intravenous Bisphosphonates
Nance and colleagues conducted one of the first investigations using an IV bisphosphonate, specifically pamidronate, which was given every four weeks for six months. Although BMD improved in incomplete ASIA impairment scale D patients at the end of the trial and marginally improved at the 6-month follow-up in complete SCI patients, benefits were not maintained at one year [69]. These findings were supported by a later study by Bauman et al. [70].
Zoledronic acid (ZA) is an agent in the treatment of osteoporosis for individuals after SCI which works by suppressing activity of the osteoclast. This pharmacologic intervention has been shown to regulate enzyme activity (farnesyl diphosphate synthetase) more effectively than other bisphosphonates and thus produces more robust effects in BMD for the general population [71, 72]. Research has been completed in patients with subacute SCI while further research is ongoing to determine the effectiveness of ZA in those with acute SCI.
Four small investigations have explored the use of ZA in subacute SCI. The first was a double-blind placebo-controlled trial by Shapiro et al. who examined the effect of intravenous (IV) ZA in 17 patients with ASIA impairment grade A or B SCI given at 10–12 weeks post injury [73]. Four subjects received 4 mg IV ZA, four received 5 mg ZA, and the remaining nine received placebo. Both the intertrochanteric region and the cortical shaft in the ZA-treated group maintained BMD near baseline values up to 12 months, while BMD declined in the control group. In contrast, the femoral neck showed an increased BMD from baseline to six months, but fell back to baseline by 12 months in the ZA group.
In the second trial, Bubbear et al. completed a randomized, open-label study of 14 patients with acute SCI, all of whom received either 4 mg IV ZA or standard medical treatment within three months (mean of 58 days of injury) [74]. Neurological levels of participants ranged from C4 to L3 and included 11 complete (five experimental, six control) and three incomplete subjects (two experimental, one control). Twelve months after the study of drug administration, the ZA-treated subjects had significantly higher BMD than the controls in three areas: 3 % for the lumbar spine (p < 0.05), 12 % for the total hip (p < 0.05), and 11 % (p < 0.05) at the greater trochanter. The 5 % increase for the femoral neck was not significant. In the ZA group, BMD at the total hip and greater trochanter was maintained from baseline, but the area of the femoral neck still lost 10 % of the original BMD. In both of the above studies, pharmacologic intervention did not occur until 2–3 months post injury, when a notable amount of bone mass has already been lost. Furthermore, neither study evaluated BMD at the distal femur and proximal tibia, two high-risk areas for sublesional fractures after SCI.
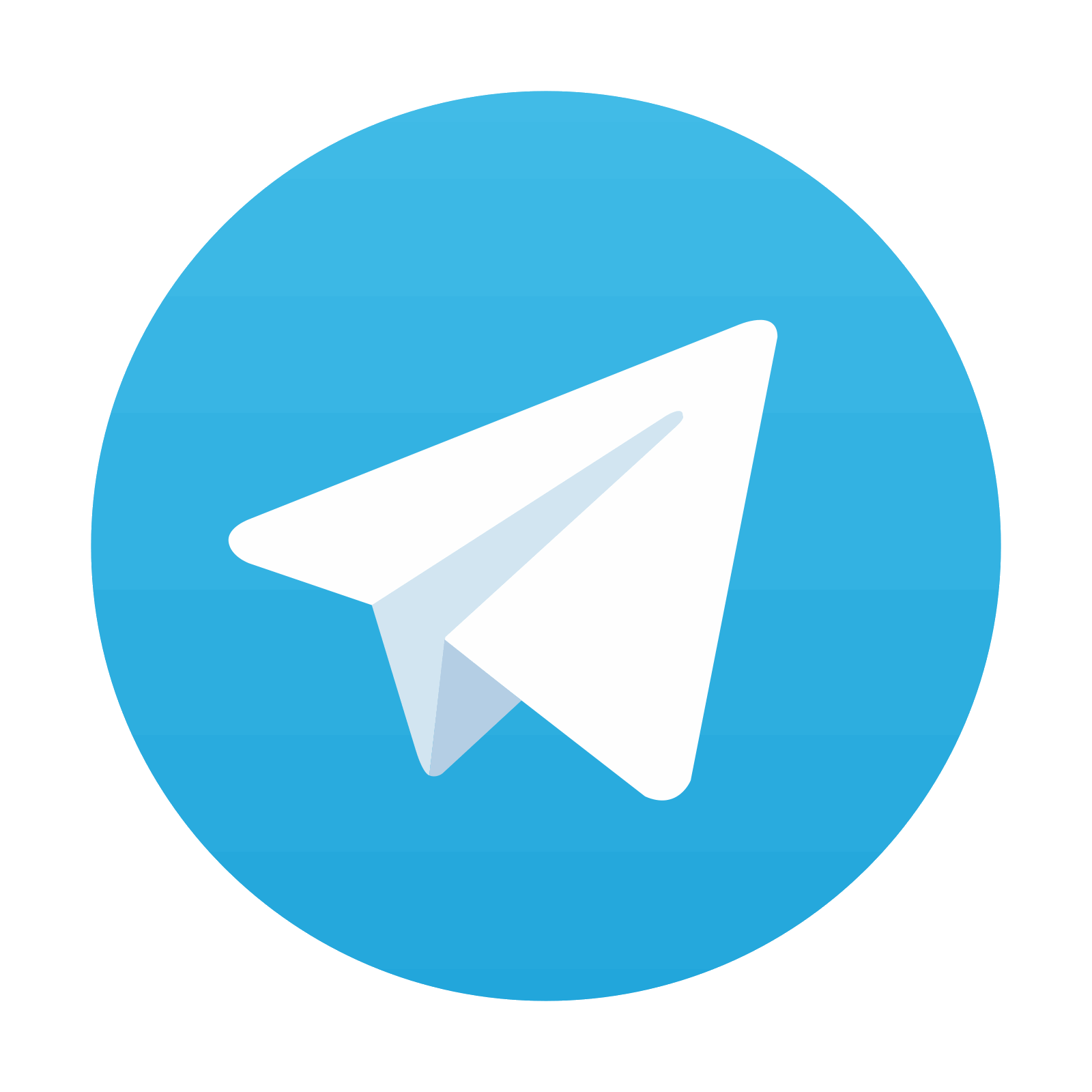
Stay updated, free articles. Join our Telegram channel
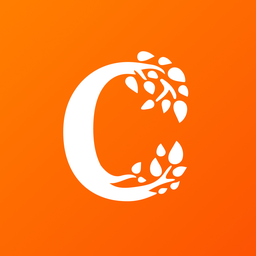
Full access? Get Clinical Tree
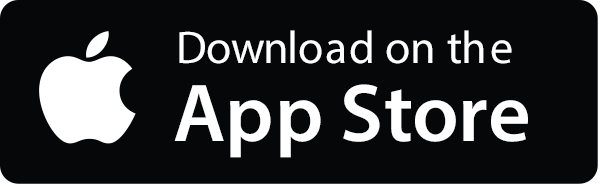
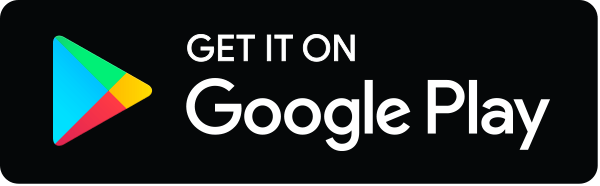