Brand 1
Brand 2
Brand 3
Brand 4
Powder components
40 g
40 g
40 g
40 g
PMMA (polymer)
88.85 %(w/w)
15.00 %(w/w)
89.25 %(w/w)
Polystyrene/MMA copolymer
75.00 %(w/w)
MMA/PMMA copolymer
83.55 %(w/w)
Benzoyl peroxide (initiator)
2.00 %(w/w)
0.5–1.6 %(w/w)
0.75 %(w/w)
Sulphate barium (radio-opacifier)
9.10 %(w/w)
10.00 %(w/w)
10.0 %(w/w)
Zirconium dioxide (radio-opacifier)
15.00 %(w/w)
Liquid components
18.37 g
20 ml
20 ml
20 ml
MMA (monomer)
98.215 %(w/w)
99.26 %(w/w)
97.40 %(v/v)
97.25 %(v/v)
N,N-dimethyl-p-toluidine
0.816 %(w/w)
1.96 %(w/w)
2.62 %(v/v)
2.75 %(v/v)
(accelerator)
0.002 %(w/w)
75 ± 15 ppm
75 ± 10 ppm
Hydroquinone (stabilizer)
15–20 ppm
Other monomeric additives
Ethyl alcohol
0.945 %(w/w)
Ascorbic acid
0.022 %(w/w)
Chlorophyll (colour additive)
0.002 %(w/w)
The polymerising process of the cement occurs as a result of the reaction between the initiator in the polymer powder and accelerator in the monomer. These act together to form a complex which produces benzoate and amine radicals. These two radicals then initiate polymerisation of the monomer [11]. A radiopacifier, added to the powder component, enables the surgeon to view the cement in vivo. This process transforms the initial thick liquid to a soft deformable material and finally to a rapidly hardening cement with an associated increase in temperature due the exothermic polymerisation which can exceed 80 °C. The cement sets through the polymerisation of the monomer, which concurrently dissolves and softens the polymer particles. The set mass consists of the polymer matrix uniting the undissolved but swollen original polymer granules. The degree of polymerisation is affected by the following: (1) the amount of accelerator and initiator in the powder and liquid monomer; (2) wetting caused by the monomer mixing with the powder; (3) the type of mixing used, (4) the pro-chilling of the monomer; and the presence of oxygen.
Physical and Mechanical Properties of the PMMA
The physical and mechanical characteristics of the acrylic bone cement were determined by the ISO 5833–1992 standard [12] (Table 10.2). The liquid and powder mixing procedure should be influenced by various factors in order to modifier the properties. These factors include the amount of the ingredient, the temperature and humidity of the mixing environment, the type of sterilisation used and the type of mixing (hand, centrifugation, or vacuum mixing) used to prepare the cement; as well as the surgical installation used in the mixing process.
Table 10.2
Physical and mechanical properties
ISO-5833 | |
---|---|
Dough time | 5 ± 1.5 min |
Setting time | 3–15 min |
Exothermic temperature | <90 °C |
Compression strength | 70 MPa |
Tensile strength | 50 MPa |
Tensile modulus | 1.8 GPa |
Physical Properties
Cement viscosity is increased by the addition of fibres, greater molecular weight of the polymer, solubility of the polymer in the monomer, variation in the powder composition or bead size distribution, and the temperature of the cement components. Pre-chilling the cement components increases the setting time and reduces the viscosity of the cement, as compared to cement components which are stored at room temperature prior to mixing. In contrast, mixing of bone cement under vacuum generally decreases the setting time. At the time mixing, the components are usually hand mixed in a bowl. However, with the use of vacuum mixing or centrifugation after mixing, the cement porosity and pore size can be reduced to improve the mechanical properties of the cured cement [13–16]. Greater monomer evaporation may occur if the applied vacuum is too great during vacuum mixing.
Poor monomer wetting in the powder can occur if: (1) the powder is insoluble or only partially soluble in the liquid MMA monomer; (2) an inadequate amount of MMA monomer is mixed into the powder; or (3) the free volume is lowered due to tighter packing of powder. On the other hand, styrene copolymers may have better wetting properties due to a higher free volume which allows for faster monomer diffusion rates [17].
High temperatures of the polymerisation process can cause evaporation of the monomer leading to microporosity in the curing cement. There are a number of factors that affect the maximum exothermic temperature. The following may contribute to a higher cement polymerisation temperature: (1) a large cement mass; (2) a cemented device with a low conduction heat;(3) a cemented device that is not cooled before implantation; (4) lack of irrigation at the implant site; (5) a greater amount of monomer mixed into the powder; or (6) increased levels of accelerators or initiators which may form radicals initiating rapid polymerisation [18].
Microporosity in the bulk cement may result form the following: (1) monomer evaporation during the exothermic reaction and/or leaching of the unreacted monomer [19]; (2) flow and wetting during mixing with the beads leading to air entrapment; (3) CO2 formation due to a benzoyl peroxide reaction with the accelerator; (4) turbulent cement flow during the insertion of the implant into the cement; (5) the mixing method used to assemble the bone cement components.
Mechanical Properties
The implant-cement-bone interfacial strengths are also considered risk factors [20]. Implant-cement interfacial loosening may result from: (1) cement fracture or poor implant-cement bonding due to foreign matter; (2) inadequate coverage at the implant-cement interface [21]; (3) amount of mechanical interlocking; or (4) a lack of chemical bonding at this interface. More specifically, the inadequate cement coverage at the interface may be caused by: (1) shrinkage of the cement due to polymerisation; (2) poor mechanical interlocking strength between the implant and cured bone cement [22]; or (3) an increase in the bone cement viscosity over time leading to poor contact between the cement and implant.
Cement-bone loosening may result from: (1) cement fracture; (2) formation of gaps at the interface; or (3) tissue failure. More specifically, the gaps may form due to: (1) bone resorption; (2) foreign material at the cement-bone interface such as bone particles or blood [23]; (3) shrinkage of the cement after implantation; (4) low cement pressurisation during implantation [24]; or (5) movement of the implant before hardening of the cement. For gaps caused by shrinkage, the shrinkage is greater as the porosity is decreased.
A fatigue fracture of the cement is a result of a cement stress which exceeds the fatigue limit of the bone cement. High cement stress may be due to an applied stress or a residual stress, cement modulus, implant loosening or poor cement bonding with the implant or with the bone. Other causes of cement fracture include; fibrous membrane formation between the bone and cement, improper cement mantle thickness (a layer too thin or too thick), lamination of the cement due to the presence of blood or other body fluids, poor canal preparation or areas of increased stress (e.g. the presence of a pore or a sharp corner of an implant). High stress applied to the bone cement may be caused by: (1) Increased patient weight or activity; (2) lack of constraint; (3) adverse implant size and orientation; or (4) inadequate bone cement mantle. The latter three are related to the quality of the tissue and the applied surgical technique. A weakness may also be caused by bone resorption or by disease.
Cement mechanical properties are affected by the level of stress at a specific site. This is influenced by: (1) irregular trabecular bone; (2) porous implant coatings; (3) sharp edges on the implant; or (4) a defect in the bulk cement such as a pore or additives. More specifically, localized stress caused by porosity and inclusions (e.g. additive agglomeration, redio-opacifiers and antibiotics) are perhaps the greatest factor affecting cement fatigue properties. The addition of agglomerates (e.g. redio-opacifiers) may also play a similar and significant role in cement fracture.
Biological Properties of the PMMA
All biomaterials must be biocompatible. PMMA cements are considered biocompatible despite the toxic potential of the bone cement monomer and the heat generated during the exothermic polymerisation.
Cellular Reactions
Initially, the major problems of PMMA bone cement are related to the temperature increase during the polymerisation and the release of residual monomer after polymerisation. PMMA is non toxic, but the residual monomer (MMA) can cause an irreversible deterioration of the cells [25, 26]. After 15 min of polymerisation, there is a residual monomer of approximately 3–5 %. This percentage may decrease up to 1–2 % with time [25]. Haas et al. [19] measured the residual MMA content to be 3.3 % after 1 h, 2.7 % after 24 h and 2.4 % after 215 days under storage in an ambient air environment. According to Schoenfeld et al. [27], the toxicity of the monomer disappears after 4 h. In our study of cement fragments which were harvested at the time of prosthetic revisions 48–78 months after implantation, there was no apparent toxic effect of the cement on the fibroblasts (L929) and human osteoblasts [28]. However, there may be variable reactions to PMMA depending of the cells involved.
PMMA is not cytotoxic with regard to human fibroblasts in vitro. However, it can stimulate proliferation and protein synthetic activity [29]. The increased proliferation of fibroblasts in response to PMMA exposure can be associated with an increased production of collagen and chemical mediators at the bone-cement interface [30, 31]. Chemical mediators, such as prostaglandin E2 (PGE2) and other cytokines (interleukine-1), have been shown to mediate inflammation, as well as induce cell division and differentiation [32, 33]. Fibroblasts have previously been implicated in the inflammatory response. Therefore, it is possible that they are responsible for the recruitment of inflammatory cells at the bone-cement interface via release of chemical mediators such as PGE2.
Monocytes and macrophages are significant agents of the inflammatory reaction. The principal function of the tissue macrophage is phagocytosis and the secretion of cytokines and growth promoters. PMMA particles induce macrophages to secrete protein and to express mRNA of the proinflammatory cytokines, interleukin-1β (IL-1β), interleukin-6 (IL-6), tumour necrosis factor alpha (TNF-α), PGE2, proteinases, collagenases and oxygen metabolites. Other factors expressed include chemokines such as macrophage-activating and chemotactic protein 1 (MCP-1) as well as macrophage inflammatory protein (MIP) which may be linked to osteolysis [34–43]. Horowitz et al. described a dose-dependent release of arachidonic acid metabolites by murine macrophages induced by PMMA particles [44].
The osteoclast is a multinucleated cell which carries out the unique and highly specialized function of lacunar bone resorption. The osteoclast belongs to the mononuclear phagocyte system which consists of various cell types including monocytes, macrophages, Kupffer cells and microglia. A common feature of all these cells is their avid and efficient ability to carry out phagocytosis. Most studies have focused on the effect of biomaterial particle phagocytosis on the function of these cells and the observation that specific types of particle enhance the release of mediators thus stimulating osteoclastic bone resorption [45–47]. In addition, it has been shown that macrophages after having phagocytosed these particulates are capable of osteoclast differentiation [48]. Wang et al. [49] found that osteoclasts having phagocytosed PMMA wear particles exhibit normal lacunar bone resorption. As well, the phagocytosis of PMMA particles does not appear to compromise the response of osteoclasts to calcitonin or to the ability to carry out lacunar resorption, an observation that remains controversial. PMMA particles can inhibit osteoblast activities causing a decrease in cellular proliferation and collagen synthesis.
Local Tissue Reactions
PMMA bone cement is generally well tolerated and bony tissue, generally, flourishes on it’s surface [29, 43]. However, there is evidence of the inflammatory potential of bone cement [50]. The tissue reaction around bone cement has several phases. Initially, there is a necrosis of the bone tissue and marrow to a depth of 5 mm depth related to the surgical wound and polymerisation. Next, there is a phase of cicatrisation lasting up to 6 months followed by a tissue granulation which develops over a period of 2 years. This tissue granulation is a characteristic of the chronic inflammation. The cement is then surrounded by a layer of fibrous tissue [51–54] and occasionally by a varying thickness of fibrocartilage [55, 56],.. Albrektsson [57] reported a 59 % reduction in the in growth of cortical bone into titanium bone chambers 1 month after cement application. Morberg et al. [58, 59] reported as well a decreased bone formation around cemented tibias, being 21 and 31 % lower than the non-cemented contralateral tibias after 3–11 and 32–55 weeks, respectively.
Osteonecrosis
Cell necrosis may occur because of the following: (1) monomer toxicity; (2) the high temperature of cement polymerisation; (3) pressure necrosis; (4) osteolysis caused by wear debris generation; or (5) the impairment of blood circulation in the bone caused by reaming and by the presence of cement [4]. Bone cement has been shown to decrease bone metabolism possibly causing a lower revascularisation [60, 61].
The production of heat at the bone-cement interface during the cement polymerisation in vitro is between 60 and 90 °C [62–64] and in vivo between 40 and 50 °C [65, 66], both depending on the thickness of the cement. The effect of this heat generation on bone was studied by Lundskog [67] who concluded that the exothermic polymerisation did not add to the surgical trauma and had no influence on bone generation. Lee et al. [68] found that the leakage of monomer was very low after the curing. Likewise, Sund and Rosensuist [69] stated “the effect of polymerisation heat and monomer toxicity are probably much less important than the trauma effected by blocking of the normal medullar blood supply”. Rhinelander et al. [66] who noted a maximal temperature of 55 °C with the placement of thermometers at the bone-cement interface, concluded that thermal necrosis from cement polymerisation is not a significant factor. Furthermore, after direct contact with acrylic cement, the delicate trabeculae of cancellous in the metaphysis contained healthy appearing osteocytes after 6 weeks.
Osteolysis
Bone surrounding an implant may undergo osteolysis leading to loosening and a decrease in cancellous bone strength. This may result in a weakening of the cement fixation or the formation of a gap between the cement and bone. Acrylic cement fragments are engulfed by eosinophilic histocytes which stimulated enzymatic release leading to bone resorption [70, 71]. In addition, bone cement particles could accelerate foreign body deterioration of articulating polyethylene inserts [72, 73]. The initial event can be either disintegration of bone cement or deterioration of the articulating surface. Phagocytosis and the development of foreign-body granulomas lead to osteolysis of the anchoring bone; thus disintegration or deterioration are enhanced accelerating the progress of osteolysis [51].
Formation of Fibrous Membrane
The formation of fibrous tissue is caused by the toxicity of the monomer release as well as the heat production of the polymerisation causing a chronic inflammation and eventual osteonecrosis and an osteolysis. It is a significant factor which induces the micromovements and the loosening of surgical implants. The thickness of the fibrous membrane around PMMA cement was of 40 μm and 60–70 μm after 1 and 4 weeks respectively in the tibiae diaphysis [53, 74]. In the human femur, the thickness was measured at 20–300 μm at 11 months to 7 years [55] and at 3–5 mm long-term. [54]
Implant Loosening
Revision of a cemented orthopaedic prosthesis may be necessary when pain occurs due to either the movement of the prosthesis, a bone fracture, bone cement fracture or prosthesis fracture. More specifically, these complications may result from prosthesis-cement, or cement-bone interfacial loosening or micromotion due to cement fracture or cement creep. Loosening of the prosthesis and fracture of the cement may lead to increased wear and bone cement particle formation. Those particles approximately less than 5 μm in size are phagocytosised by macrophages which become activated and directly or indirectly cause bone remodelling and osteolysis [14, 75–78]. However, PMMA particles ingested by macrophages cannot be degraded by lysosomal enzymes [45]. The final result is cell death leading to tissue necrosis and chronic inflammation [79]. For the femoral stem, the lower viscosity bone cement had a revision rate 2,5 times greater when compared to the use of higher viscosity cements. Additionally, a lower modulus cement had a revision rate that was 8.7 greater than the higher viscosity cements [80]. In general, revisions are required between 3.6 and 22.8 years following a total hip prosthesis. The most frequent periods of revision are either during the first 3 years or after 8 years postoperatively [81]. The aseptic loosening of the prosthesis is the principal cause of revision, implicated in: 73–74 % of the total cases [82, 83]. Subcritical debonding associated with mechanisms of cyclic fatigue crack growth are particularly relevant considering that these systems will experience over 1,000,000 physiological loading cycles per year, and are expected to survive a minimum of 10–15 years. In these terms, it is critical to understand the progressive debonding of prosthesis-PMMA cement interface [84].
Secondary Reactions
Systemic and Cardiovascular Reactions
Methylmethacrylate (MMA) is very volatile and is rapidly cleared from body through the lungs resulting in a local concentration that remains very low [85, 86]. MMA monomers escaping from the implanted polymerising cement have been associated with a decrease in both systolic blood pressure and arterial oxygen tension [87] and possibly cardiac arrest. [88] However, many studies have not confirmed this direct correlation between the concentration of MMA and blood pressure, heart depression or vasodilatation [86, 89, 90]. Circulatory disturbance during hip implantation may be primarily due to either the “implantation syndrome” or to the blockage of pulmonary circulation by fat, bone marrow and entrapped air rather than MMA monomer. Release of MMA could cause a drop in the partial pressure of arterial oxygen leading to an increased heart rate [91, 92]. The possible metabolic pathway of MMA monomer is that the residual monomer is converted to methylacrylic acid rather than methylester. The methylacrylic acid, as a coenzyme A ester, is a normal intermediate in the catabolism of valine and the existence of an enzyme system would permit methylacrylic acid to enter a normal pathway, leading to carbon dioxide formation. Over 80 % of an administered dose of MMA is expired as carbon dioxide within 5–6 h [90].
Sensitising
While MMA is considered to be relatively immunologically inert, it can induce phagocytosis, the activation of macrophages and giant cells as well as the migration of inflammatory mononuclear cells [13, 14, 52, 74, 78]. Jensen et al. [52, 74] showed that MMA is extremely active in a guinea-pig maximisation test. The hospital personnel who repeatedly handle coring acrylic bone cement are potentially at risk of developing a delayed sensitivity [93]. Bengston et al. [94] reported that patients having received a cemented hip prosthesis had increased levels of anaphylatoxines which can contribute towards circulatory and respiratory disturbances. In contrast, Kanerva et al. [95] have found allergies to MMA to be rare in a study of patients between 1974 to 1992 (4 patients: a orthodontist, 3 dental technicians).
Improvement of the PMMA
The objective of the development of PMMA bone cement is to improve the biocompatibility, to diminish the temperature of polymerisation, to eliminate the generation of wear debris and fatigue fractures, as well as to increase the elastic modulus. Therefore, efforts to improve PMMA bone cement have proceeded in two main directions: (1) to change the composition and (2) to improve preparative techniques.
Tertiary aromatic amines are used as accelerators for the benzoyl peroxide (BPO) initiated MMA polymerisation. A complex series of reactions occurs between BPO and the amine, and free radicals are produced that initiate the polymerisation process [96, 97]. Several types of amine accelerators, such as dimethyl aniline and its derivatives, have been used in the polymerisation of MMA by the amines/BPO initiator system. Their relative efficiency as accelerators and their activating effects on the rate of polymerisation have been reported [96, 97]. Several workers have studied bone cement properties using a number of N,N-dimethyl-p-toluidine derivatives, such as, 4-dimethylaminobenzyl methacrylate, and 4-dimethylamino phenethyl alcohol [96–99]. Bone cement products containing residual monomer and amine have been reported in preparation where the amines/BPO molar ratio is outside the equimolar range [11]. Other study showed that MMA polymerisation in the presence of tir-n-butylborane used as the cure initiator does not occur too rapidly, and the high temperature during polymerisation is lower than that of conventional bone cement. The application time is short enough for clinical use, namely, within 10 min. As for the physical properties, it has a 3 % lower elastic modulus and greater ductility than the conventional cement [100].
Several workers have added particles or fibres to PMMA bone cement to improve the biocompatibility et the mechanical properties. The fibre reinforced bone cement possessed significantly greater stiffness and displayed poor intrusion characteristics [101–103]. A number of attempts have been made at filling a PMMA matrix with hydroxyapatite and tricalcium phosphate particles, and with bioactive glass [104–106]. The short-term results obtained are encouraging and suggest that the chemical nature of the bone/bioactive materials interface is very important relative to osteoconductivity [107]. For instant, PMMA bone cement can be used only to effectuate mechanical fixation for prosthesis or to physically fill bone defects. It however, does not exhibit the functions of osteointegration, biofixation, nor bioresorption.
Calcium Phosphate Cement (CPC)
Chemical Compositions and Crystallisation of the CPC
Calcium phosphate cements can be handled in paste form and set in a wet medium after precipitation of calcium phosphate crystals in the implantation site. Depending on the products involved in the chemical reaction leading to the precipitation of calcium phosphate, different phases can be obtained with different mechanical properties, setting times and injectability. Numerous components can enter the chemical reaction leading to calcium phosphate precipitation. More than 100 different of calcium orthophosphate cements were used to determine the compressive strength and the diametric tensile strength after storage. The setting was carried out on more than 15 formulations. These cements could be divided into four classes: dicalcium phosphate dihydrate, calcium and magnesium phosphates, octocalcium phosphate, and non-stoichiometric apatite cements [108, 109]. The calcium and phosphate compounds in Table 10.3 were often used to make the CPC. Moreover, adjuvants such as chitosan, lactic acid and glycerol are added to improve the injectability of the cement, and accelerators such as Na2HPO4, sodium phosphate, sodium succinate, and sodium chondroitin sulphate to accelerate its setting time.
Table 10.3
Calcium and phosphate compounds
Name | Abbreviation | Formula | Ca/P | Solubility | Acidity | Stability |
---|---|---|---|---|---|---|
Monocalcium phosphate monohydrate | MCPM | Ca(H2PO4)2 · H2O | 0.5 | |||
Dicalcium phosphate anhydrous | DCPA | CaHPO4 | 1.0 | +++++ | +++++ | + |
Dicalcium phosphate dihydrate | DCPD | CaHPO4 · 2H2O | 1.0 | +++++ | +++++ | + |
Octacalcium phosphate | OCP | Ca4H(PO4)3 | 1.33 | ++++ | ++++ | ++ |
Amorphous calcium phosphate | ACP | Ca9H(PO4)6 | 1.3–1.5 | +++ | +++ | +++ |
Tricalcium phosphate | TCP | Ca3(PO4)2 | 1.5 | ++ | ++ | ++++ |
Hydroxyapatite | HAP | Ca10(PO4)6(OH)2 | 1.67 | + | + | +++++ |
Tetracalcium phosphate | TTCP | Ca4(PO4)2O | 2.0 |
The hardening process of CPC is complex and involves the dissolution of solid particles in the liquid, precipitation of HAP from the solution, and the reaction and diffusion on the particle surface. Under ideal conditions, continuing dissolution of the reactions supplies calcium and phosphate ions to the solution, while HAP formation depletes these ions. This process drives the solution composition to an invariant point, which is the intersection of the solubility curves for these two reactants. The pH is about 7.8, but this process is affected by many parameters, such as the component and the particle sizes of the solid phase, presence of HAP seed and properties, aqueous liquid, etc.
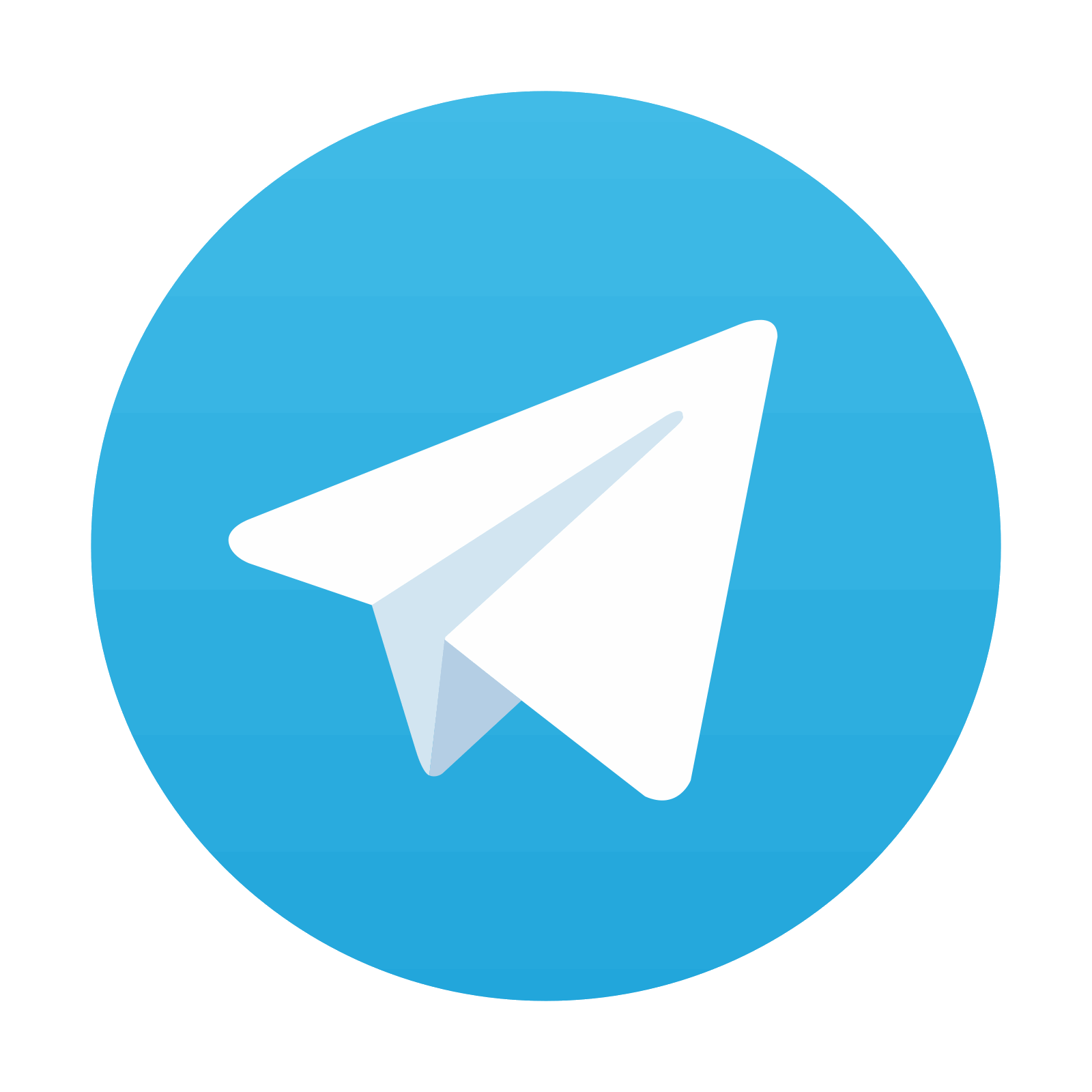
Stay updated, free articles. Join our Telegram channel
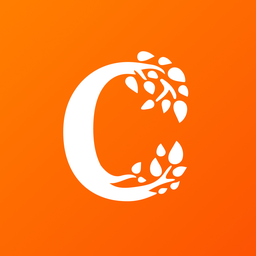
Full access? Get Clinical Tree
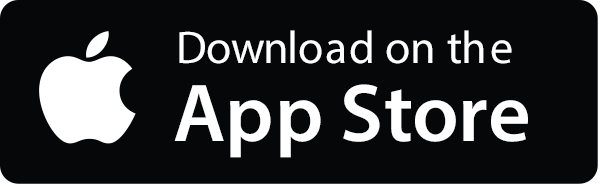
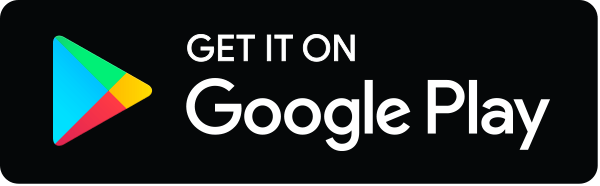