Oral rehydration and electrolyte balance
• Traditional oral rehydration solutions are made according to World Health Organization guidelines for the prevention and treatment of dehydration during diarrhea or gastroenteritis. For these medical conditions, effective delivery of oral fluid replacement is best achieved by a solution that is low in carbohydrate (2 %, or 2 g/100 ml) and provides electrolytes in concentrations of sodium 50–80 mmol/l and potassium 10–30 mmol/l (e.g., Pedialyte®)
• Newer sports drinks have become available with a higher sodium content (~30 mmol/l) to help replace sodium lost in sweat
Replenishment of energy reserves
Sports drinks
• Usually a carbohydrate-rich solution (6–8 % carbohydrate), containing mainly sodium (10–25 mmol/l) and potassium (3–5 mmol/l). Drinks with additional electrolytes (calcium, chloride, magnesium, potassium) and micronutrients (chromium) are also available
Sports gels
• A highly concentrated carbohydrate source (65–70 %) in an easily consumed and quickly digested gel form
• Usually has substantially higher carbohydrate concentration than sports drinks. Use with caution as idiosyncratic gastrointestinal distress may occur
• Some gels also contain other compounds, such as medium-chain triglycerides (MCTs) and small amounts of caffeine (~20–40 mg)
Sports bars
• Compact source of carbohydrate and protein in a bar form. Generally low in both fat and fiber, although there are exceptions. Some are fortified with micronutrients (typically containing about 25–50 % RDA per bar of various vitamins and minerals)
Liquid meal supplements
• Carbohydrate-rich, moderate protein, low-fat powder (or liquid) for mixing with water or milk
• Generally intended to supply a balance of macronutrients and micronutrients
Minimizing oxidative damage: antioxidants
• Classically, vitamins C and E as well as other micronutrients such as zinc and selenium. Newer additions include l-carnitine, extracts of certain fruits/vegetables, coQ10, and teas
Muscle repair
Whole protein and protein hydrolysates
• Whey (isolate and concentrate)
• Casein
• Egg
• Soy
Amino acids
• Essential amino acids
• Branched-chain amino acids (leucine, isoleucine, valine)
• Glutamine
• Creatine
• Hydroxy beta-methylbutyrate (HMB)
Carbohydrates
• See above
Immune system support
• Amino acids (glutamine)
• Vitamins (ascorbic acid, vitamin D)
• Plant extracts (Andrographis paniculata, ginseng, ashwagandha)
• Omega-3 fats (from fish oil, krill oil)
12.4 Rehydration
Fluid loss is inherent in exercise. Indeed, with prolonged activities such as running or cycling, athletes may lose 1–3 l of body water per hour. These losses may in turn produce a dehydration-associated loss of body weight and impairment of cardiovascular and thermoregulatory homeostasis [1]. The loss of vascular fluid volume may then result in intracellular water being drawn out of muscle cells [2], an obvious detriment to performance. Clearly, although it is often overlooked as a postexercise dietary supplement or ergogenic, it is of the utmost importance that the appropriate fluid replacement volume be considered along with the attendant need of proper electrolyte balance.
12.4.1 Water and Sodium
The health benefits of plain water have often been touted and are not disputed here. However, the use of plain water as a sport rehydration beverage may not be ideal under certain circumstances. For example, copious water ingestion may cause relative dilution of the serum sodium level [3], which in turn may cause a decrease in thirst sensation and an increase in urine output, stalling the rehydration process. A better option for ensuring a proper plasma volume is to use a sodium/water solution [4]. In fact, it has been previously demonstrated that when adequate fluid is available, rehydration is achieved more rapidly when the solution sodium content (0.3–0.7 g NaCl per liter of water) is greater than sodium lost via sweat [5, 6]. This level of rehydration sodium is recommended by both the American College of Sports Medicine [7] and the National Athletic Trainers’ Association [8]. Potassium may also be added to the rehydration solution after exercising, although experimental evidence has not confirmed substantial improvement in intracellular rehydration [9, 10].
Because of the combination of exercise-associated water losses and the expected increase in urine production during the postexercise consumption period, it is generally recommended that consumption of 150 % or more of the weight lost may be required to achieve euhydration within the short-term postexercise period (i.e., 6 h) [5, 11, 12]. With such impressive fluid losses and the need for considerable fluid consumption, the palatability of a fluid replacement drink is of no small concern. In fact, research suggests that drinks with a slight citrus taste are especially effective at preventing the quenched sensation following ingestion of water alone [13]. It is no coincidence, then, that the popular sports drinks in the American marketplace (e.g., Gatorade, Powerade, Accelerade) all offer a citrus flavor. However, those popular beverages contain sodium concentrations (0.4–0.5 g NaCl per liter of water) at the low end of that recommended for rapid and complete restoration: 0.3–0.7 g NaCl per liter of water. When rapid rehydration is the primary goal during the postexercise period, a better choice may be found in a number of specially concentrated solutions (e.g., Endurox R4, Powerbar Recovery, HEED, etc.). Perhaps also worthy of consideration are the clinical oral rehydration fluids (used to treat diarrhea and dehydration), although a potential drawback of these more concentrated saline solutions is an unpalatable taste, which could affect the total volume consumed. It is also possible to achieve the recommended sodium intake by consuming water with food. Because food consumption slows gastric emptying, the rehydration process is similarly slowed.
In summary, complete and rapid postexercise rehydration requires a special fluid intake plan. Consumption of 150 % of fluid losses allows complete fluid restoration. To enhance the process, a supply of palatable drinks should be made available after exercise. Flavored and lightly sweetened drinks are generally preferred and can contribute to achieving carbohydrate intake needs as well. The main drawback of commercial (nonclinical) rehydration beverages lies in their relatively low sodium content. As previously noted, replacement of sodium lost in sweat is important for maximizing retention of ingested fluids. A sodium content equivalent to 0.3–0.7 g NaCl per liter water may be necessary for optimal rehydration.
12.4.2 Carbohydrate–Electrolyte Solutions
With the rise in consumption of carbohydrate-containing sports drinks has come increased scrutiny of their value to the exercising athlete. In particular, there were concerns regarding the potential for slowing the gastric emptying time. However, subsequent studies have demonstrated that dilute (6–8 %) solutions of glucose, glucose polymers (maltodextrin), and other simple sugars all have suitable gastric emptying characteristics for the delivery of fluid and moderate amounts of carbohydrate substrate [14]. As previously noted, most widely available sports drinks provide a low level of sodium (0.3–0.7 g NaCl per liter of water) and thus may not be the ideal rehydration beverage (depending on the athlete’s needs) despite their carbohydrate value and palatability.
12.4.3 Glycerol
Glycerol (also called glycerine) is a three-carbon molecule that forms the structural backbone of triglycerides and phospholipids. It is technically a sugar alcohol, but it can be converted to glucose in the liver without a major change in plasma glucose or insulin levels. Because of this conversion, the direct energy yield of glycerol is similar to that of glucose, although it is not a major energy source when carbohydrates are freely available. Glycerol is a common ingredient in many nutritional bar products.
Glycerol has a somewhat unique physiological role in terms of its ability to influence water balance. When glycerol is added to rehydration beverages, it blunts the natural decrease in antidiuretic hormone (ADH) release that occurs with rehydration. Sustained ADH activity results in greater retention of body fluids and, potentially, enhancement of postexercise rehydration and thermoregulation during exercise in hot, humid environments [15]. This property has not gone unnoticed clinically, and glycerol is commonly utilized in patients with glaucoma to reduce intraocular pressure.
The use of glycerol in postexercise rehydration supplements has demonstrated improved short-term hydration but not reliable improvement in athletic performance [15–17]. However, the dosage of glycerol is perhaps important, as some studies that utilized lowered amounts (0.5 g/kg versus ~1.0 g/kg body weight) did not improve rehydration [18].
In summary, the potential for glycerol-enhanced rehydration is best attempted at a dosage of 1 g/kg body weight with adequate water ingestion as described in the previous section. It is considered a safe, effective supplement, and it has not been shown to elicit negative effects with regard to postexercise recovery or subsequent exercise performance [19].
12.4.4 Fluid Balance in American Football Players
12.4.4.1 David Chorba
American football players face some unique challenges regarding thermoregulation during preseason training, particularly linemen with relatively high body mass compared to that of other athletes. A small body surface area/body mass ratio and above-average body fat percentage increase the metabolic load of exercise. These athletes are less able to dissipate heat, which is magnified by wearing up to 15 lb. of protective gear.
Sweating rates are similar for football athletes and cross-country runners exercising in the same environmental conditions when adjusted for body size. However, longer practice durations, two-a-day preseason sessions, and insulating equipment create a higher overall sweat production in football players (>2 l/h and in some athletes >3 l/h) [12]. Daily fluid losses from sweating during two-a-day practices can average more than 9 l/day, with some athletes losing more than 14 l/day [12]. This amount of fluid loss requires 125–150 % replacement (during and after practice) to account for urine losses that occur with fluid ingestion [20]. Such a large fluid intake can cause mild symptoms associated with hyponatremia (although rare in football) if too much plain water is ingested and dietary sodium intake is inadequate during preseason meals.
With such large fluid losses through sweating, the electrolyte balance must be considered in football athletes. Sodium losses from sweating range from 20 to 80 mmol/l [21] and have been documented up to 110 mmol/l in professional football players [22]. Normally, sodium and chloride are reabsorbed by sweat glands after excretion. However, this ability does not increase proportionately with the sweating rate, and the electrolyte concentration in sweat increases [23, 24]. A day’s sweat loss of 9.4 l with a sodium concentration of 50 mmol/l equates to >10 g of sodium depletion [12]. Significant sodium depletion can occur with excessive sweating, especially in individuals with poor aerobic conditioning and/or suboptimal heat acclimatization. Some football athletes are prone to large, acute sodium losses during sweating (5.1 g vs. 2.2 g during a 2.5-h practice) and despite consuming sodium-containing fluids on the field are more likely to experience associated heat cramps [25]. These athletes may benefit from ingesting fluids with sodium in addition to making sure they consume sufficient dietary sodium (1.5 g day) [26] during meals.
Despite a significantly larger body mass and heat-trapping protective gear, collegiate football athletes during summer preseason two-a-day practices do not experience the continuous core temperature increases seen in distance runners training in the same environment [27]. Frequent breaks (averaging 71 s, documented for football athletes) cause core temperature fluctuations that may provide the necessary recovery time to dissipate heat. Despite a more intermittent nature of training, however, football athletes were found to be less well hydrated than their endurance-training counterparts. Distance runners may hydrate more frequently throughout the day in preparation for long runs without access to fluids; however, the intermittent nature of football practice should leave adequate opportunity for players to rehydrate.
It is imperative that football athletes properly acclimate to the environment and physical stresses imposed by intense preseason training in order to decrease the risk of adverse health events such as heat exhaustion and heat stroke. Acclimatization requires progressive adaptations to the environment, the physical training, and the protective equipment worn by the athlete. In an effort to decrease the risk of heat-related injury, the National Collegiate Athletic Association (NCAA) has implemented a heat-acclimatization protocol during preseason training [28]. The protocol requires that protective equipment during practice begins with helmets only and progressively increases to full pads. In this way, exercise intensity is also progressed, as more demanding activities usually require additional protective gear. In addition, exercise duration is progressed to two-a-day practices performed on alternate days, with at least 3 h of recovery time between sessions. The new NCAA model has been shown to improve exercise-heat tolerance and heat acclimatization in Division I football players [29]. An added benefit of proper heat acclimatization is the improved ability to reabsorb sodium and chloride, resulting in a 50 % lower sweat sodium concentration for a given sweating rate [23].
Fluid losses greater than 2 % of body weight have been shown to impair cognitive/mental function and athletic (aerobic) performance in temperate to hot conditions [30–32], and losses greater than 3 % increase the risk of heat-related illness [8, 32]. Most individuals replace only half of the fluids lost during exercise voluntarily [33]. Therefore, it is important that all athletes engaging in prolonged exercise in the heat are assessed for hydration status and follow a water replacement schedule. Individual hydration protocols should be developed that consider the athlete’s sweat rate, sport dynamics, environmental factors, acclimatization state, exercise duration and intensity, and individual preferences [8, 32]. The American College of Sports Medicine and the National Athletic Trainers’ Association provide guidelines to assist in designing customized fluid replacement programs [8, 32].
A simple, effective method to assess hydration status involves tracking body weight before and after practices. An athlete should be weighed first thing in the morning after voiding for 3 days consecutively (more days for women due to menstrual cycle changes) to establish the baseline, euhydrated (normal) state [32]. General recommendations to ensure appropriate hydration from the National Athletic Trainers’ Association [8] include consuming 17–20 oz. of water or a sports drink 2–3 h before exercising and 7–10 oz. 20 min prior to exercise. Fluid replacement during exercise should attempt to match sweat and urine losses, with no more than 2 % total body weight loss. This generally equates to 7–10 oz. every 10–20 min of exercise but is highly dependent on the individual athlete, as described above. After exercise, athletes should be weighed (after urination) and then consume 450 ml (15 oz.) for 125–150 % of body weight loss in pounds, ideally within 2 h after exercise. The addition of 0.3–0.7 g NaCl/l is acceptable to stimulate thirst and increase voluntary fluid intake for shorter sessions; it should also be considered during initial training days in hot weather, during exercise lasting more than 4 h, and when inadequate access to meals is anticipated. For intense sessions or those lasting more than 45–60 min, consuming a sports drink containing 6–8 % carbohydrate can speed replenishment of glycogen stores and delay fatigue, as well as contribute to the palatability of the beverage.
12.4.5 Exercise-Associated Hyponatremia
12.4.5.1 Rita Chorba
At the South African Ironman Triathlon, an experienced 34-year-old male ultramarathoner completing the race in just under 12.5 h was brought to the medical tent near the finish line with mild confusion, sleepiness, and difficulty concentrating and maintaining conversation [34]. His hands and face were swollen, and he complained of feeling ill. Testing revealed an absolute weight gain during the race of 3.8 kg (8.4 lb.), which, after consideration of fuel oxidation and fluid release during the race, approximates at least 5 kg (11 lb.) excess fluid. Blood analysis revealed a serum [Na+] of 127 mmol/l, which was decreased from a before-race value of 143 mmol/l. The athlete was subsequently diagnosed with exercise-associated hyponatremia (EAH).
Despite medical management including sodium replacement, the athlete had initial difficulty producing enough urine to decrease the fluid overload and required overnight hospitalization until his serum [Na+] normalized and he had excreted 4.6 l of urine. Only then was the athlete permitted to resume drinking fluids. It was later discovered that the athlete had ingested 750 ml fluid per hour during the cycling component (more than 6 h in duration), 750 ml early in the run, and then “as much as possible” throughout the remainder of the run, as he thought his ill feelings were signaling dehydration. This amount of fluid ingestion was contradictory to the briefing given to all athletes before the race, where it was recommended that no more than 500–800 ml/h be ingested during the race. In fact, these new recommendations resulted in only this one athlete being treated for hyponatremia in the South African Ironman (0.2 % of entrants), compared to previous races exhibiting rates of 18 and 27 % [2, 3] Studies of Ironman athletes have revealed an inverse relation between post-race body mass and [Na+], as those who lost less weight during competition (or gained weight) had the lower plasma [Na+] [34–36].
Commonly known as water intoxication, EAH is a dilutional hyponatremia caused by consuming too much plain water during periods of prolonged exercise [4]. Although drinking excessive fluids normally stimulates urine production, this mechanism is impaired during sustained endurance exercise, increasing the potential for hyponatremia [37, 38]. EAH can occur when excessive ADH is released, causing fluid retention and maintaining decreased serum [Na+] with subsequent cell edema. Although symptomatic EAH was previously described in terms of sodium loss, more recent studies confirm significant fluid overload as the primary independent factor, with sodium loss playing a secondary role in the pathogenesis of the condition [34, 37, 39, 40].
Sodium loss through sweating combined with dilution of extracellular sodium due to excessive ingestion of hypotonic fluids (plain water) causes serum sodium concentrations to fall, as sodium is drawn into the unabsorbed water in the intestines. Whereas moderate loss or dilution (serum sodium < 135 mEq/l) can lead to headaches, confusion, nausea, and muscle cramps, concentrations below 125–130 mEq/l can create more life-threatening situations [41–43].
Severely decreased serum sodium concentration creates a significant osmotic imbalance, causing water to rush into brain and lung cells. The subsequent swelling may cause seizures, coma, pulmonary edema, or death. A number of factors lead to EAH [37].
Athlete related | Event related |
---|---|
Low body mass index | Prolonged exercise (>4 h of activity) |
Female gender | Extreme cold conditions |
Slow performance pace, poor conditioning | Unusually hot conditions |
Race inexperience | High availability of drinking fluids (frequent aid stations) |
Excessive fluid intake behaviors | |
Weight gain during the event | |
Altered renal excretory capacity (use of NSAIDs or diuretics, renal disease) |
Although continuous, high-intensity exercise for more than 1 h can deplete fluid and sodium levels, athletes at risk for hyponatremia most commonly include ultramarathoners and triathletes, as well as marathoners who take more than 4 h to complete the race [44].
Previous recommendations for the prevention of EAH included drinking ad libitum (according to thirst) no more than 400–600 ml/h (13–20 oz.), the lower limit for slower, lighter persons in cool weather, and the upper limit for faster, heavier athletes in warm weather [37, 45]. As stated previously, similar guidelines were employed in an Ironman Triathlon of significantly longer duration with a dramatically decreased incidence of hyponatremia [34]. That said, we do not promote strict adherence to the above fluid quantities as it is well known that athletes demonstrate a substantial interindividual variation in sweat rates and renal excretory capacity during exercise. The best approach is for athletes to drink according to their thirst levels before, during, and after exercise. In addition, athletes should closely monitor their body weight during exercise, expect a ~2 % decrease under certain conditions, and never gain weight (due to fluid ingestion) during exercise. While not foolproof, having a drinking schedule prepared for various competitive situations (that have been tested in practice conditions) provides an effective strategy for reducing the incidence and severity of EAH.
Although it seems prudent to recommend increased sodium intake during prolonged exercise, it might not be necessary and might not be effective in decreasing the risk of EAH. Recent guidelines by the Institute of Medicine suggest that the daily adequate intake of Na+ (1.5 g/day) is appropriate for physically active people, considering that the sodium intake in a Western diet averages 2.3–4.7 g/day [26]. In another investigation, during the South African Ironman Triathlon [46], ingestion of an additional 3.6 g of sodium (15 tablets, equal to 9 l of Gatorade) during competition along with usual sports drinks and food was not necessary to preserve serum [Na+] despite more than 12 h of high-intensity activity, nor did supplementation affect any other performance, physiological, or psychological variables collected. The one exception was the athlete with hyponatremia described previously [34] who was part of the placebo group for the current study [34]. However, he was also the only athlete to demonstrate substantial weight gain during competition from overhydrating. It is postulated that during acute Na+ loss, contraction of the extracellular volume or Na+ release from intracellular stores may act to buffer Na+ loss until it is replenished during a subsequent meal [26]. Consumption of electrolyte-containing sports drinks may be able to decrease the severity of EAH [47], although not all studies have shown that their consumption prevents development of EAH in the presence of overdrinking [48, 49]. This may be due to the fact that most electrolyte-containing sports drinks are hypotonic solutions, containing less than 135 mmol/l sodium, and can therefore contribute to further sodium dilution in the presence of fluid excess (see Fig. 12.1).
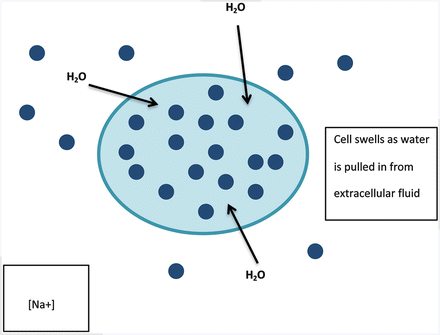
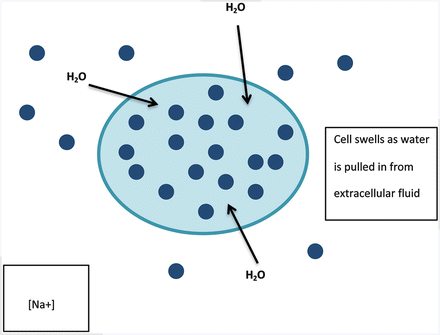
Fig. 12.1
Illustration of sodium dilution due to excess fluid levels
In summary, exercise-associated hyponatremia is a condition of decreased serum sodium concentration occurring during or up to 24 h after prolonged endurance exercise and is most often associated with excessive fluid consumption. EAH can lead to serious consequences, including pulmonary and cerebral edema. Persons at greatest risk, including deconditioned or race-inexperienced athletes participating in multi-hour events, are advised to drink according to thirst and to prepare race-day fluid replacement strategies that mirror fluid losses measured under practice conditions.
12.4.6 What Are Electrolytes?
Electrolytes are minerals that become ions in solution and acquire the capacity to conduct electricity. Proper electrolyte balance in the body is essential for normal functioning of cells. Loss of body water and subsequent alterations in electrolyte balance can impair cardiovascular and thermoregulatory function. In terms of exercise performance, the most important electrolytes are sodium, chloride, and potassium. Sodium is the primary positive ion (cation) in the extracellular fluid and helps regulate acid–base balance, nerve conduction, blood pressure, and muscle function. Chloride is the primary negative ion (anion) in the extracellular fluid and works in tandem with sodium to regulate nerve impulse conduction and body water balance. During exercise, the body loses fluids and electrolytes (mainly sodium and chloride) via sweat. The resulting decrease in blood volume increases the relative sodium and chloride concentrations in blood and triggers the thirst mechanism. Potassium is the primary cation in intracellular fluid and helps maintain electrical activity in nerves, skeletal muscles, and the heart. Potassium also aids carbohydrate metabolism by enhancing glucose transport and glycogen storage. Finally, calcium and magnesium are cationic electrolytes that play important roles in the regulation of muscle contraction and enzymatic reactions. Because their losses in sweat are minimal, they are usually not included in hydration solutions.
Generally, electrolyte replacement is not needed during short bouts of exercise, as sweat is approximately 99 % water and less than 1 % electrolytes. Adequate water, in combination with a nutrient-dense, well-balanced postexercise diet, can restore normal fluid and electrolyte levels in the body. However, replacing electrolytes may be beneficial during continuous activity of longer than 90 min, particularly in a hot, humid environment. Most research shows there is little evidence of physiological or physical performance differences between consuming a carbohydrate–electrolyte drink versus plain water during exercise lasting less than 1 h [32].
12.5 Muscle Fuel Energy
An important goal of the athlete’s everyday diet is to provide the muscle with substrates to fuel the training program and achieve optimal performance, recovery, and long-term adaptation. Carbohydrate and fat constitute most of the exercising muscle’s energy fuel, with protein typically playing a minor role. For example, even the leanest of athletes store enough fat (>100,000 kcal) to fuel exercise, but by comparison the same athlete’s carbohydrate stores are limited (~2,500 kcal). It is important to recognize that the initial concentration of stored muscle carbohydrate can dictate performance in a variety of exercises. Therefore, the restoration of muscle energy fuels, particularly carbohydrate, is a principal concern for optimal recovery and subsequent exercise performance.
12.5.1 Carbohydrate
Skeletal muscle stores the simple carbohydrate glucose in highly branched glycogen granules. Stored glucose is then released during muscle contraction for both anaerobic and aerobic energy productions. Approximately 500 g of carbohydrate is stored in the muscle of an average-sized male with an additional 100 g stored in the liver and available for release into the blood. Muscle glycogen is an essential fuel source for sustained moderate- to high-intensity aerobic and anaerobic exercise metabolism [50, 51]. Therefore, replenishment of depleted muscle glycogen levels after strenuous exercise is paramount to complete recovery. Glycogen restoration is of utmost importance for preparation for a subsequent training or competition bout of exercise. Furthermore, failure to restore muscle glycogen between exercise sessions results in compromised training and competition capacity and contributes to symptoms of overtraining [52].
Classic work by Costill et al. found that individuals consuming a high-carbohydrate diet could replenish their postexercise muscle glycogen to normal pre-exercise concentrations within 24 h [53]. These investigators reported that the consumption of 600 g of carbohydrate per day resulted in proportionately greater muscle glycogen restoration during the 24-h period after exercise. However, consumption of more than 600 g provided no additional benefit. When dietary carbohydrate was inadequate (i.e., <150 g in 24 h) during successive days of intense exercise, there was a gradual reduction in muscle glycogen stores and deterioration in performance [52]. Thus, when insufficient carbohydrates are consumed during the 24-h postexercise period, suboptimal glycogen levels result particularly over the course of several successive days of intense training. By applying the appropriate postexercise carbohydrate restoration practices described below, athletes can avoid such a decrement in muscle carbohydrate stores.
12.5.1.1 Glycogen Storage Immediately After Exercise
Recent research has focused on the most effective means of promoting glycogen replenishment during the early hours of exercise recovery. Postexercise synthesis of glycogen occurs in a biphasic fashion, with the initial, rapid phase occurring in the first 30 min and the subsequent, slower phase of resynthesis occurring until muscle glycogen levels are fully restored [54]. Fatigued muscles are highly sensitive to nutrient activation following exercise, with the sensitivity declining over time. Therefore, to maximize the rate of muscle glycogen repletion, an athlete must initiate carbohydrate restoration supplementation immediately after the completion of exercise.
Resynthesis of muscle glycogen is twice as rapid if carbohydrate is consumed immediately after exercise in contrast to waiting several hours [55]. This rapid rate of synthesis can be maintained if carbohydrate is consumed throughout the hours following exercise. For example, supplementing carbohydrate at 2-h intervals at a rate of 1.5 g/kg body weight appears to maximize resynthesis for a period of 4 h after exercise [55]. Carbohydrate provided immediately after exercise can be in the form of solid or liquid provided the glycemic index (GI) is high and the presence of other macronutrients minimized so as not to affect the rate of gastric emptying, intestinal absorption, or glycogen storage [56–58]. Practical considerations, such as the availability and appetite appeal of foods or drinks and gastrointestinal comfort, may determine individual carbohydrate choices and intake patterns. Review of appropriate carbohydrate types is provided later in the chapter. Intriguingly, postexercise muscle glycogen synthesis may be enhanced with the addition of protein and certain amino acids. Furthermore, the combination of carbohydrate and protein has the added benefit of stimulating amino acid transport, protein synthesis, and muscle tissue repair. Both of these topics are discussed later.
12.5.2 Sustained Postexercise Glycogen Storage
Maehlum et al. [55] and Ivy et al. [55] have demonstrated that the postexercise rate of glycogen storage plateaus at carbohydrate ingestion rates of 1–2 g/kg. Consumption of more than 2 g/kg provides no additional benefit and may result in gastrointestinal distress. Investigators have determined that providing carbohydrate at 1.5 g/kg every 2 h (in either liquid or solid form) after exercise resulted in a near-maximal rate of glycogen resynthesis over the first 4 h of recovery [55, 57]. Other scientists have also reported sustained, rapid resynthesis of muscle glycogen stores from similar rates of carbohydrate intake (i.e., 1.5 g/kg every 2 h), particularly when high-GI carbohydrate foods are consumed [59]. Over a 24-h period, this equates to ingesting carbohydrate at approximately 7–10 g/kg relative to body weight per day. Again, practical considerations, such as the availability and appetite appeal of foods or drinks and gastrointestinal comfort, may determine ideal carbohydrate choices and intake patterns.
12.5.3 Glycogen Supercompensation
Because of the paramount importance of muscle glycogen during intense, prolonged exercise as well as exercise of an anaerobic nature, methods for maximizing initial, pre-exercise levels and replenishing the glycogen stores on a day-to-day basis have been studied extensively [50, 60]. The provision of adequate carbohydrate during the recovery process can result in “supercompensation” of muscle glycogen storage. In other words, more glycogen can be stored than prior to the previous exercise bout. Postexercise carbohydrate feeding promotes glycogen supercompensation by increasing blood concentrations of glucose and insulin. Insulin promotes muscle carbohydrate storage by increasing blood flow through the muscle and by regulating the synthesis of glycogen in the muscle. Specifically, insulin regulates the synthesis of glycogen in two steps: first, by increasing the transport and uptake of glucose into muscle cells and, second, by increasing the intramuscular enzymes involved in glycogen synthesis and decreasing the enzymes involved in degradation [61]. Exercise is important in this process as muscle contraction results in an increase in insulin stimulation of glucose transport into the muscle cell [62, 63] and indirectly enhances insulin-mediated activation of key enzymes of the glycogen synthesis pathway [64].
Bergstrom and Hultman [65] first observed that glycogen synthesis occurred most rapidly in muscle depleted of its related glycogen stores. They also found that consumption of a high-carbohydrate diet for 3 days would elevate the glycogen concentration of the muscle above normal and that this phenomenon was observed only in the muscle that was previously glycogen depleted by exercise. Later, Sherman and colleagues provided findings for a modified glycogen supercompensation regimen in which a 5-day workout taper was accompanied by a moderate (50 % of caloric intake) dietary carbohydrate intake for the first three taper days followed by 3 days of higher carbohydrate intake (i.e., 70 % caloric intake) [66]. In a follow-up study, these investigators determined that pre-exercise muscle glycogen levels could be maintained at high levels despite daily training if carbohydrate was consumed at a rate of 10 g/kg/day. More recently, Bussau and associates described a 1-day glycogen supercompensation regimen [67].
12.5.3.1 Differences Between Simple Carbohydrates
Fructose, sucrose, and glucose are common dietary carbohydrates. However, the physiological responses to the consumption of these “simple” sugars differ considerably. For example, the rise in blood glucose and insulin following ingestion of fructose is significantly lower than that following ingestion of glucose [59]. This is due to the necessary conversion of fructose to the more readily utilized glucose by the liver. Researchers have demonstrated that ingestion of glucose and high-GI carbohydrate are twice as effective for restoring muscle glycogen [59, 68]. Blom and colleagues suggested that the differences between the glucose and fructose supplements were due to the ways the body handles these sugars [59]. Fructose metabolism takes place predominantly in the liver, whereas most glucose appears to bypass the liver to be stored or oxidized by the muscle [51]. Thus, carbohydrate supplement products containing glucose (dextrose) or glucose polymers are more effective for restoring muscle glycogen after exercise than supplements composed predominantly of fructose. Many postexercise supplement drinks contain glucose or glucose polymers, and, despite its name, the common food sweetener high-fructose corn syrup (HFCS) contains a significant percentage of glucose (~50 %).
12.5.4 Protein
Recent research demonstrates that ideal postexercise meals/beverages should contain both carbohydrate and protein. This combination promotes an accelerated rate of muscle glycogen storage possibly by activating the glycogen synthesis pathway by two mechanisms [69, 70]. First, it raises the plasma insulin level beyond that typical of a carbohydrate supplement, which may augment muscle glucose uptake and activate glycogen synthase, the rate-limiting enzyme for glycogen synthesis. Second, the increase in plasma amino acids that occur as a result of protein consumption may activate glycogen synthase through an insulin-independent pathway, thus having an additive effect on the activity of this enzyme. Continued supplementation at 2-h intervals can maintain an active glycogen recovery for up to 8 h after exercise. The combination of carbohydrate and protein provided immediately after exercise is also ideal for stimulating protein synthesis and tissue repair. This is due to the additive effect of insulin and amino acids on the enzymes controlling amino acid transport, protein translation, and protein degradation. Moreover, research has demonstrated that appropriate nutrient supplementation after exercise can have a significant impact on subsequent physical performance [69].
In summary, general guidelines for postexercise carbohydrate and protein intake are the following: (1) During the first 3 h after exercise, ingest two or three mixed meals providing approximately 400–600 kcal (each). (2) For strength/power athletes, a carbohydrate/protein ratio of 2:1 is recommended. (3) For team sport athletes, a 3:1 ratio is recommended. (4) For endurance athletes, a 4:1 ratio is recommended. (5) Make sure the first postexercise meal includes high-GI carbohydrates (dextrose, maltodextrin) and rapidly digesting protein (whey protein concentrates, hydrolysates). (6) Be sure to provide more energy for larger athletes and/or for higher volumes of training. For endurance athletes engaged in repeated high-volume training, the recommended daily target for carbohydrate consumption of 10–13 g/kg relative to body weight per day is sufficient.
When the period between exercise sessions is short (<12 h), the athlete should begin carbohydrate–protein intake as soon as possible after the first exercise bout to maximize the effective recovery time between sessions. There may be some advantages in meeting carbohydrate–protein intake targets with a series of snacks during the early recovery phase, but during longer recovery periods (1–2 days), the athlete should plan their timing of high-GI, carbohydrate-rich feedings according to what is most practical and comfortable for their individual situation.
12.5.5 Lipids
Athletes rarely ingest dietary fat to improve their performance and recovery, but there are several specialized lipids that may have unique effects on human physiology. Medium-chain triglycerides (MCTs) are fatty acid constituents of coconut and palm kernel oils. They have an important action as energy-providing molecules, in particular for individuals with intestinal malabsorption syndromes [71]. Indeed, from the medial perspective, MCTs have been shown to be helpful for nutritional support in a number of conditions, including the specialized nutritional need of some infants, the chronically ill, and surgical patients [72–74]. For almost a decade, MCTs have been promoted as useful for weight loss and improving athletic performance. However, to date, most studies have shown that MCTs are ineffective in this regard. For example, researchers have investigated the role of MCTs in the enhancement of long-chain fatty acid oxidation, preservation of muscle glycogen, and the ability to improve overall exercise performance [75–77]. In general, results were unimpressive. More importantly, some studies even reported that exercise performance was impaired in subjects taking high doses of MCTs. This reduction in performance was associated with an increase in gastrointestinal problems, such as intestinal cramping. For these reasons, use of MCTs during the postexercise period cannot be justified at this time.
12.5.5.1 Conjugated Linoleic Acid
Conjugated linoleic acid (CLA) is a group of isomers of the essential fat linoleic acid. CLA is naturally found in a number of animal meats, eggs, and dairy products. CLA has drawn interest in the sports nutrition community because of a reported ability to increase lean body mass and reduce body fat [78–80] and more recently improve immune function [81]. Specifically, Song and associates reported that 12 weeks of supplementation (at 3 g/day) with a 50:50 blend of the cis-9, trans-11 and trans-10, cis-12 isomers significantly decreased levels of the pro-inflammatory cytokines tumor necrosis factor-α (TNFα) and interleukin-1β (IL-1β) while simultaneously increasing levels of the anti-inflammatory cytokine IL-10 [81]. Although this study needs to be confirmed by other research studies, it indicates that CLA may be useful for enhancing recovery by limiting exercise-induced inflammation and soreness. It should be noted that although the trans-10, cis-12 isomer appears to be responsible for the fat-lowering effects of CLA [82, 83], some studies have reported that supplementation with this purified isomer in high doses (i.e., 2.6 g/day) may increase insulin resistance, lower high-density lipoprotein (HDL) cholesterol, and elevate biomarkers of oxidative stress in obese men with metabolic syndrome [84]. In contrast, the aforementioned 50:50 blend of CLA does not appear to have these adverse effects, even after prolonged use (i.e., up to 2 years).
12.5.6 Other Muscle Substrates
12.5.6.1 Creatine
Creatine monohydrate, or methyl guanidine-acetic acid, is presently one of the most popular ergogenic sport supplements. Endogenously, creatine is an amino acid-like compound produced in the liver, kidneys, and pancreas from arginine, glycine, and methionine. Creatine is a nonessential dietary compound that is both endogenously synthesized, primarily in the liver from the amino acids arginine, glycine, and methionine, and naturally ingested through an omnivorous diet by consuming meat, fish, and poultry. However, because of typically having lower total muscle creatine contents, vegetarians possess a greater ability to uptake creatine when compared to their omnivorous counterparts [85]. Endogenously synthesized creatine is released from the liver into the bloodstream and then taken up by muscle fibers predominately by way of a sodium-chloride-dependent creatine transporter (CrT). Creatine ingested through supplementation has been observed to be absorbed into the muscle exclusively by means of CrT [86].
The major rationale of creatine supplementation is to increase the intracellular pool of total creatine (creatine + phosphocreatine). Once taken up into the muscle, approximately 65 % of creatine is phosphorylated by the enzyme creatine kinase to form phosphocreatine (PCr). The remaining 35 % exists in the muscle as free (unphosphorylated) creatine. The intracellular level of phosphocreatine (PCr) plays a significant bioenergetics role during the immediate energy system, typically referred to as the ATP–PCr system, which is predominately active during high-intensity, short-duration, and repeated bouts of exercise. During this type of exercise, depletion of intracellular PCr stores occur, yet intracellular adenosine triphosphate (ATP) concentration is maintained by a freely reversible reaction, catalyzed by creatine kinase, in which PCr phosphorylates adenosine diphosphate (ADP) to replenish ATP stores. This bioenergetic system provides the impetus to increase the duration of high-intensity exercise from only a few seconds to up to 10–15 s. It should be noted, however, that PCr levels within the muscle are almost 3–4 times more abundant than intramuscular ATP stores. Therefore, the PCr supply is sufficient in providing a temporary ATP source until other bioenergetic systems reach maximal rates. Another role that PCr functions is to act as an acid–base buffer to prevent declines in intracellular pH since the creatine kinase reaction utilizes a hydrogen ion. Muscular contraction can be negatively impacted by metabolic acidosis due to marked reductions in intramuscular pH.
Hundreds of clinical trials have been conducted on creatine monohydrate supplementation as a means of enhancing exercise performance. In general, approximately 70 % of the clinical trials have found beneficial effects, particularly during short, repeated bursts of high-intensity activity. The most common creatine supplementation dosing regimen that has indicated an increase in intracellular PCr and performance is 20 g/day (divided into four equal doses each day) for 5–7 days and is usually followed by a maintenance phase of 5 g/day. While this approach seems more appropriate for someone who is using creatine to prepare for a sporting even 1–3 weeks following initiation of supplementation, for those who intend on supplementing on a daily basis for an extended period, a loading phase is not necessary to achieve positive results [87]. Studies examining pre- vs. postexercise consumption of creatine are in their nascent stages. Consequently, until a general consensus is reached regarding timing of creatine consumption relative to exercise, the authors recommend “bracketing” the exercise session with multiple doses (i.e., ingest 2.5 g pre-exercise and another 2.5 g postexercise).
It should be noted that at least one study has reported that longer-term (e.g., 3–6 months) creatine supplementation reduced CrT activity and muscle creatine uptake [88]. These findings imply that it would be undesirable to consume high doses of creatine for an extended amount of time. Thus, a 1 month washout period following 1–3 months of supplementation would be prudent to avoid downregulation of the creatine transporters. Furthermore, it would also be advisable to avoid consuming extremely high doses of creatine, as this would likely downregulate the CrT over time. As a result, some experts suggest creatine cycling (i.e., 4 weeks of use followed by a 4-week break) based on the premise that once muscle creatine stores are elevated they can remain so (with performance enhanced) for an additional 4–5 weeks without creatine supplementation.
Another issue worth considering is since creatine uptake will most likely differ in regard to weight and muscle mass, ingesting creatine at an absolute dose may not be best but rather at a relative amount based upon body weight (approximately 0.7 g/kg of body mass) or fat-free mass (approximately 0.1 g/kg of fat-free mass). In light of these dosing strategies, some researchers have found no improvement via supplementation; thus the term nonresponders has been established. Since intracellular creatine rather than PCr levels determine the regulation of creatine uptake and CrT activity [89], it is likely that this variability is due to the regulatory process of the CrT that controls both the influx and efflux of creatine across the muscle cell membrane. Another possibility is that “nonresponders” already have high levels of intramuscular creatine stores. Although responses are quite variable from person to person, the ingestion of creatine typically produces an average 2–5 lb. greater gain in muscle mass and 5–15 % greater increases in muscle strength and power compared to control (or placebo) subjects [90–92].
Efforts have been made to attempt to determine the most effective means to enhance muscle creatine uptake. Because it is also known that the uptake and storage of creatine can be augmented by up to 60 % when blood levels of insulin are elevated [93], many individuals co-ingest creatine with high-GI carbohydrates (usually dextrose, maltodextrin, or fruit juice). Adding Cr with a carbohydrate source has been observed to enhance uptake, primarily through the effect of an insulin response. Additionally, research has indicated that combining creatine with sodium and/or chloride may additionally enhance Cr uptake via the manipulation of increasing the gradient in which the CrT functions [94]. Finally, it should be noted that no study has ever been published demonstrating greater benefits to strength, lean mass, or performance with creatine salts (e.g., creatine hydrochloride, creatine citrate, creatine pyruvate) compared to creatine monohydrate.
12.5.6.2 d-Ribose
d-Ribose is an organic pentose monosaccharide (five-carbon sugar) found in all living cells. Ribose (β-d-ribofuranose) forms part of the backbone of RNA. Ribose is sometimes referred to as a “genetic sugar” because of its importance in the nucleosides of RNA as well as ATP. All foods have some d-ribose, but certain substances, such as brewers’ yeast, are rich in RNA and thus are thought to be good sources of d-ribose. Ribose is related to deoxyribose, which is found in DNA. Phosphorylated derivatives of ribose such as ATP and NADH play central roles in metabolism. The cyclic monophosphates, cAMP and cGMP, formed from ATP and GTP serve as secondary messengers in some signaling pathways. However, there is a mismatch between ATP hydrolysis and synthesis rates that are associated with high-intensity exercise that lead to an overall reduction of total adenine nucleotides [(AdN) = ATP + ADP + AMP] within the myocyte. Once the ATP-derived purine molecule is lost from the muscle cell, recovery of the AdN pool must depend on the de novo synthesis and/or the purine salvage metabolic pathways, and these pathways proceed at relatively slow rates [95]. Studies have shown that repeated days of short-term, high-intensity exercise in humans led to a significant reduction in resting ATP concentration in the previously exercised muscles [96, 97]. Although the pathways for skeletal muscle AdN synthesis proceed slowly, an impactful increase in their rates occurs with ribose supplementation [98]. It has also been shown that ribose supplementation increased adenine salvage rates in resting skeletal muscle and that high-intensity exercise had no diminishing effect on adenine salvage rates in the postexercise recovery period in ribose-supplemented muscle [99].
Current thinking is that ribose supplementation would be an effective ergogenic aid by virtue of its ability to resynthesize ATP and “boost energy” based on the fact that ribose supplementation has been shown effective at increasing AdN synthesis and adenine salvage. Even though a number of studies have been performed attempting to demonstrate the role of ribose as a performance enhancer, overall the data do not support this presumption. For example, single-bout studies providing 3–32 g of d-ribose before exercise did not report improvements in anaerobic exercise capacity or adenine nucleotide breakdown [100, 101]. Also, a study in which 10 g of d-ribose was ingested for five consecutive days indicated no beneficial effect on anaerobic exercise capacity or metabolic markers [102]. A similar study involving 6 days of oral ribose supplementation (4 × 4 g doses) was shown to have no effect on maximal intermittent exercise performance or postexercise muscle ATP recovery [103]. Thus, although there may be clinical applications of high-dose (~60 g/day) d-ribose in chronic fatigue syndrome and fibromyalgia, at present the use of d-ribose as an ergogenic nutritional supplement cannot be adequately justified.
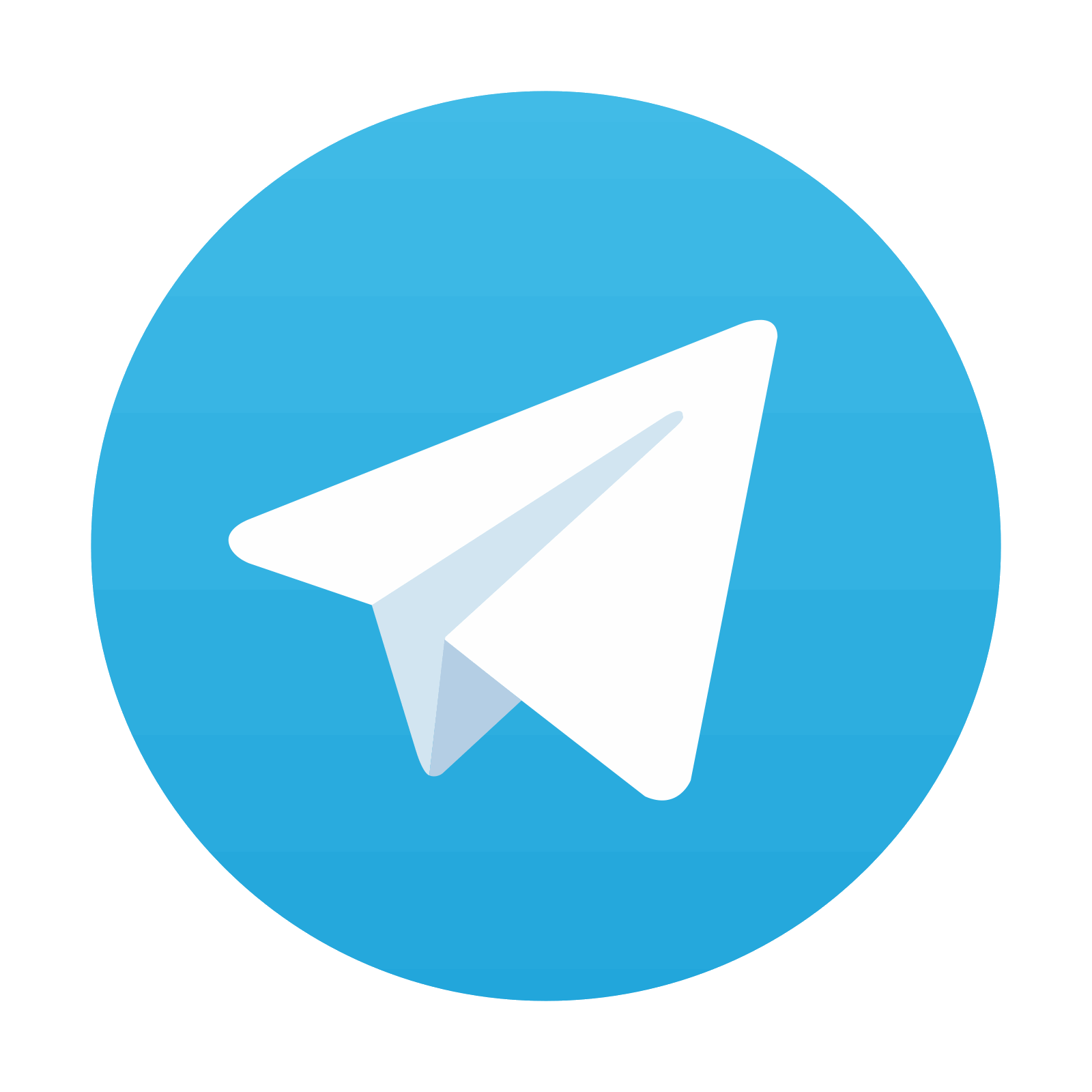
Stay updated, free articles. Join our Telegram channel
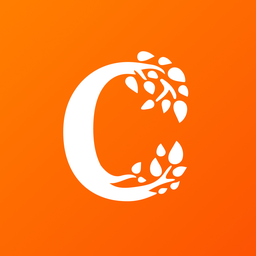
Full access? Get Clinical Tree
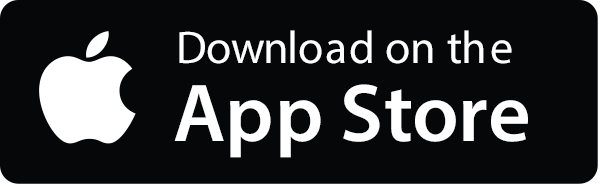
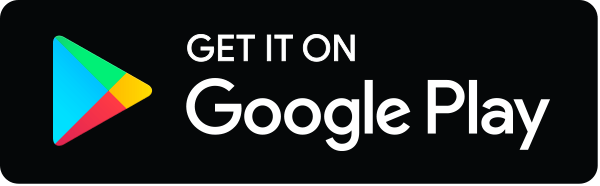