Normal and impaired fracture healing

Mechanical performance of bone
The mechanical performance of the human skeleton is secured by the constant adaptation of bone to its mechanical loading environment. Mechanical adaptation of bone is brought about by the coordinated actions of osteoclasts and osteoblasts, which are orchestrated by the most mechano-sensitive cells in bone, the osteocytes [1].
It is common knowledge nowadays that increased mechanical loading (eg, due to exercise) increases, and unloading (eg, due to bedrest) decreases bone mass, mineral content, and bone matrix protein production. This phenomenon is known as functional adaptation of bone, and it serves to obtain bones that combine a proper resistance against mechanical failure with a minimum use of material. More than 20 years ago Frost [2] postulated his mechanostat theory that predicts that there are threshold levels of mechanical strain above which adaptive bone formation is activated, and lower levels, below which bone resorption is activated. According to the mechanostat theory, bone loss takes place due to the activation of bone remodeling below a certain mechanical stimulation. Indeed, it is likely that the actual adaptation of bone to the ever changing mechanical demands takes place during the complicated process of bone remodeling [3, 4]. However, bone remodeling takes place continuously, and depending on the balance between bone formation and resorption within the newly formed osteons, bone mass can be lost or gained.
Bone remodeling
Bone is a living tissue and not some lifeless scaffold to put a plate on when fractured. Human bone contains over 15,000 osteocytes per mm3. Mouse bone contains even more than 60,000 osteocytes per mm3 of bone [4, 5].
Bone is not a static tissue. As a matter of fact, old bone is constantly being removed by osteoclasts and replenished by osteoblasts in a coordinated fashion. This process, known as bone remodeling, presumably serves to prevent, or remove microscopic damage in the matrix that occurs due to continuous “wear and tear”. Bone remodeling thus prevents so-called fatigue fractures, which occur when microdamage accumulates. The constant renewal of bone also aids the adaptation of bone to its mechanical environment. It allows the osteoclasts to remove excess bone in places bearing only relatively small loads, and the osteoblasts to add bone in places that are exposed to relatively high levels of loading.
The microscopic damage that occurs due to tissue fatigue is assumed to be the actual signal for activation of the process of bone remodeling [6–8]. Fatigue damage will likely promote osteocyte apoptosis, which attracts osteoclasts, thereby activating bone remodeling [7–11]. After activation, bone remodeling involves groups of osteoblasts and osteoclasts, which collaborate in a tightly coordinated fashion in so-called basic multicellular units (BMUs). Within these BMUs, the osteoclasts excavate a tunnel within the compact bone, or a trench along the surface of trabeculae. The osteoclasts are closely followed by osteoblasts that refill the tunnel [12]. Frequently an osteoblast buries itself within the newly formed matrix, thereby forming the new osteocytes. The entombed cells assume a stellate shape, with cell bodies positioned in lacunae in the matrix, from which slender cell processes radiate in all directions. The cell processes pass through the bone matrix via small canals, the canaliculi, in order to keep in contact with other osteocytes as well as the cells outside the bone matrix. The osteoblasts do not completely fill the tunnel that is excavated by the osteoclasts, but a space is left in the middle for blood vessels, providing the osteocytes with nutrients and oxygen. In this way, osteons are formed, containing osteocytes at a maximum of 6 cell layers deep, and a blood vessel in the middle (Fig 1.2.1-1).

Bone biology
Bone consists of cells and extracellular matrix. The extra-cellular matrix is composed of 35% organic material, and 65% inorganic material. The inorganic component is largely in the form of hydroxyapatite, which is a crystalline calcium phosphate mineral. The organic part of bone is made up of collagen and noncollagenous matrix proteins. The protein collagen is organized into polypeptide chains, and a double helix of chains and molecules that form the collagen fibrils. The collagen fibrils are grouped in bundles to produce the collagen fiber. The holes in between the fibrils are filled with proteins and mineral deposits, through which mineralization commences. Collagen type 1 is the major structural protein component of bone, and provides its tensile strength. Although small in number, the biological impact of noncollagenous proteins is important. Noncollagenous proteins include osteocalcin, osteonectin, osteopontin, phosphoproteins, thrombospondin, and bone growth factors. Osteocalcin and osteonectin possess specific calcium-binding properties and initiate hydroxyapatite crystal production during mineralization. The phosphoproteins are capable of binding to the bone-resorbing cells, the osteoclasts, and therefore are involved in the regulation of bone resorption. Bone growth factors can affect both the cells by which they are produced (autocrine effect) or other cells (paracrine effect).
The osteoclasts comprise 1% of all bone cells and originate from haematopoietic stem cells. The osteoclasts’ function is to resorb bone. The osteoblasts (5% of all bone cells) produce bone. Similar to osteocytes and bone-lining cells (94% of all bone cells), osteoblasts originate from mesenchymal progenitor cells. Bone-lining cells line the bone surfaces, while osteocytes are situated inside the bone. The orchestrated cooperation of osteoblasts and osteoclasts forms the basic multicellular unit, through which bone remodeling takes place [12]. Until around the year 2000 the function of the osteocytes was still unclear.
Mechanical loading stimulates interstitial fluid flow
Significant progress has been made in understanding how osteocytes may sense and transduce mechanical signals derived from bone loading [14–23]. These studies emphasize the role of osteocytes as the “professional” mechanosensory cells of bone, and the lacuno-canalicular porosity as the structure that mediates mechanosensing [22]. Strain-derived flow of interstitial fluid through this porosity seems to be sensed by osteocytes. The osteocytes are thereby informed about the mechanical inadequacy of the surrounding tissue. Overuse as well as disuse produces abnormal canalicular flow, which is translated into bone forming or bone resorbing signals [24, 25]. This concept facilitates an explanation of local bone gain and loss during adaptation, as well as remodeling in response to fatigue damage as processes supervised by mechanosensitive osteocytes [13].
According to Wolff′s law, bone tissue adapts its mass and structure to the prevailing mechanical loads applied, resulting from gravity and muscle function. The mechanical loads applied to the bone causes flow of interstitial bone fluid in the lacunar canalicular porosity (not in the Haversian or Volkmann channels, through which blood vessels run). The fluid flow (resulting in a fluid shear stress on the osteocytes) is sensed by the osteocytes, which communicate through the syncytium with the bone cells on the surface (bone-lining cells, osteoblasts, and osteoclasts). These cells on the bone surface in turn respond with bone resorption (osteoclasts) and/or deposition of bone (osteoblasts). In addition, stress-generated electrical potentials might be involved in bone adaptation. These streaming potentials might be sensed by osteocytes as well.
Burger and colleagues have developed a theory that links osteocyte sensing of different loading-induced canalicular flow patterns around the cutting cone and reversal zone during remodeling to the coordinated actions of osteoblasts and osteoclasts in a BMU [4, 13]. Volumetric strain in the bone around a bone multicellular unit cutting cone has been related to canalicular fluid flow [26], and the predicted area of low canalicular flow around the tip of the cutting cone was proposed to induce local osteocyte apoptosis. Mechanical loading by fluid-shear stress has been shown to promote osteocyte survival, while unloading is associated with osteocyte apoptosis [11, 27]. Osteocyte apoptosis is a likely trigger for osteoclastic bone resorption. Osteocyte apoptosis at the tip of the cutting cone would thus attract osteoclasts, leading to further excavation of bone in the direction of the loading. The model by Smit and colleagues [26] further predicts that at the base of the cutting cone and further down the reversal zone, osteocytes receive enhanced fluid-shear stress during loading. This could prevent osteocyte apoptosis, but may also stimulate the release of signaling molecules that promote the retraction and detachment of osteoclasts from the bone surface. The two mechanisms, attraction of osteoclasts to the cutting cone tip and induction of osteoclast detachment from the cutting cone base, together could explain the mechanically meaningful behaviour of osteoclasts during remodeling (see Fig 1.2.1-1).
Osteocyte morphology in different bone pathologies
Bone adapts to increased mechanical loading by an increase in bone density and to decreased mechanical loading by a decrease in bone density. The apparent difference in bone density in patients with osteopenia, osteopetrosis, and osteoarthritis can be explained amongst others by a difference in adaptation to mechanical loading. This suggests a difference in the mechanosensitivity of osteocytes in these three bone types, which might result from a difference in osteocyte morphology [28]. The possibility that osteocyte morphology might play a role in various bone pathologies was explored by using nano-CT and confocal microscopy, which demonstrated significant differences in osteocyte 3-D morphology in osteopenic, osteopetrotic, and osteoarthritic bone, ie, osteocytes in osteoarthritic bone were relatively small and elongated, whereas osteocytes in osteopenic bone were relatively large and round, and osteocytes in osteopetrotic bone were relatively small and had a round, discoid shape [29]. Osteopenic bone showed large osteocyte lacunae, whereas the osteocyte lacunae in osteopetrotic bone were smaller. The smallest osteocyte lacunae were found in osteoarthritic bone. Since the osteocyte cell body likely has a role in direct mechanosensing of matrix strain and adapts its 3-D morphology to mechanical loading for efficient mechanosensing [30], differences in cell shape might reflect differences in osteocyte mechanosensitivity, which might relate to differences in bone mineral density (BMD). The exact relationship between osteocyte morphology and bone architecture, however, is complex and deserves further study, but the relationship between osteocyte morphology and bone architecture does provide new insight and might contribute to a better understanding of the cellular processes that lead to different BMD in various bone pathologies.
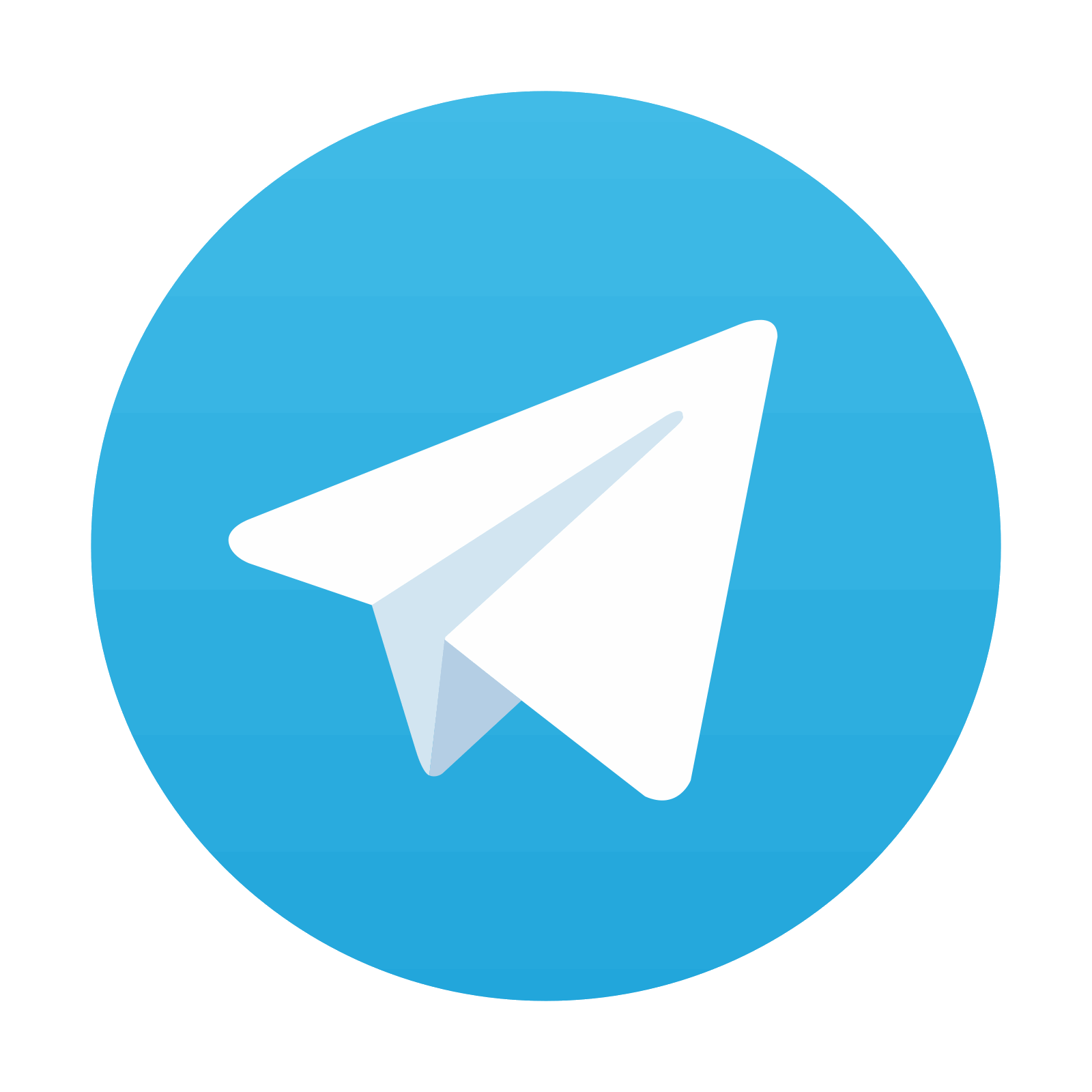
Stay updated, free articles. Join our Telegram channel
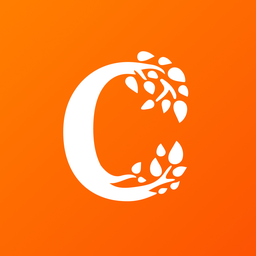
Full access? Get Clinical Tree
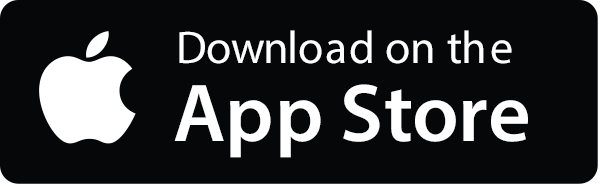
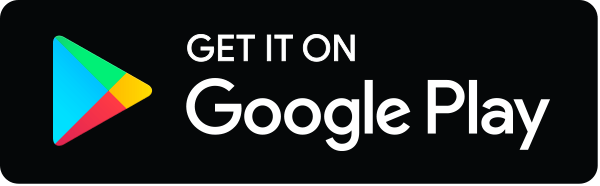