Cartilage Morphometry and Spoiled Gradient-Recalled-Echo, Driven Equilibrium Fourier Transform, Steady-State, High-Field, Phase-Sensitive, and Forward-Looking/Experimental MRI Methods
- Garry E. Gold
Articular cartilage pathology may be the result of degeneration or acute injury. Osteoarthritis is an important cause of disability in our society and is primarily a disease of articular cartilage. Acute injury to cartilage may be characterized using MRI. Whether the result of degeneration or injury, MRI offers a noninvasive means of assessing the degree of damage to cartilage and adjacent bone and measuring the effectiveness of treatment.
Many imaging methods are available to assess articular cartilage. Conventional radiography can be used to detect gross loss of cartilage, which is evident as narrowing of the distance between the bony components of the joint, but it does not image cartilage directly. Secondary changes such as osteophyte formation can be seen, but conventional radiography is insensitive to early chondral damage. Arthrography, alone or combined with conventional radiography or CT, is also mildly invasive and provides information limited to the contour of the cartilage surface.
MRI, with its excellent soft tissue contrast, is the best technique currently available for assessment of articular cartilage. Imaging of regions of cartilage damage has the potential to provide morphologic information about the region, such as fissuring and the presence of partial-or full-thickness cartilage defects. The many tissue parameters that can be measured by MRI techniques have the potential to provide biochemical and physiologic information about cartilage.
An ideal MRI study for cartilage should provide accurate assessment of cartilage thickness and volume, demonstrate morphologic changes of the cartilage surface, demonstrate internal cartilage signal changes, and allow evaluation of the subchondral bone for signal abnormalities. Also desirable would be an evaluation of the underlying cartilage physiology, including the status of the proteoglycan and collagen matrices. Conventional MRI sequences in current clinical use, however, do not provide a comprehensive assessment of cartilage and are lacking in either spatial resolution or specific information about cartilage physiology or require impractically long scan times for such assessments.
CONVENTIONAL MRI METHODS
MRI has emerged as the leading method of imaging soft tissue structures around joints. One of the major advantages of MRI is the ability to manipulate contrast to highlight tissue types. The common contrast mechanisms used in MRI are 2D or multi-slice T1-weighted, proton-density (PD)–weighted, and T2-weighted imaging, with or without fat suppression. Imaging hardware and software have changed considerably over time, including improved gradients and radiofrequency coils, fast or turbo spin-echo imaging, and techniques such as water-only excitation.
Whereas the tissue relaxation times and imaging parameters are the major determinants of contrast between cartilage and fluid, lipid suppression increases contrast between nonlipid and lipid-containing tissues and affects how the MR scanner sets the overall dynamic range of the image. The most common type of lipid suppression is fat saturation, in which fat spins are excited and then dephased before imaging. Another option is spectral-spatial excitation, in which only water spins in a slice are excited. Finally, in areas of magnetic field inhomogeneity, inversion recovery provides a way to suppress lipids at the expense of signal to noise and contrast to noise.
The type of contrast used in cartilage imaging is critical to the visibility of lesions and the signal-to-noise ratio (SNR) of the cartilage itself. Although T2-weighted imaging creates contrast between cartilage and synovial fluid, it does so at the expense of cartilage signal. The high signal from fluid is useful to highlight surface defects such as fibrillation or fissuring, but variation in internal cartilage signal is poorly depicted. These scans also are often done in 2D, leaving a small gap between slices, which may therefore miss small areas of cartilage damage.
Spatial Resolution
MRI of cartilage requires close attention to imaging spatial resolution. To see degenerating cartilage, imaging with resolution on the order of 0.2 to 0.4 mm is required. The ultimate resolution achievable is governed by the SNR possible within a given imaging time and with a given radiofrequency coil. Ultimately, a high-resolution imaging technique that combines morphologic and physiologic information would be ideal in the evaluation of osteoarthritis. Given current techniques, it is likely that a combination of a high-resolution morphologic imaging sequence with a sequence for matrix evaluation will be the most useful.
2D Fast Spin-Echo Imaging
Currently, imaging of the musculoskeletal system with MRI is often limited to 2D multi-slice acquisitions acquired in multiple planes. This is commonly done with turbo or fast spin-echo (FSE) methods. These methods provide excellent signal to noise and contrast between tissues of interest, but the inherently anisotropic voxels in these 2D acquisitions require that multiple planes of data be acquired to minimize partial-volume artifacts. For example, a typical sagittal image may have 0.3 to 0.6 mm in plane resolution but a slice thickness of 3 to 5 mm. FSE techniques show excellent results in the detection of cartilage lesions ( Figs. 8-1 and 8-2 ) . These methods provide excellent depiction of structures in the the imaging plane, but evaluation of oblique or small structures across multiple slices can be challenging. For these reasons, 3D acquisitions with thin sections are appealing.







3D Gradient-Recalled-Echo Techniques
Traditional 3D gradient-recalled-echo (GRE) methods have the potential to acquire data with more isotropic voxel sizes but suffer from a lack of contrast compared with spin-echo approaches. High accuracy for cartilage lesions has been shown with 3D-SPGR (spoiled gradient-recalled-echo) imaging. There are two main disadvantages to this approach: lack of reliable contrast between cartilage and fluid that outlines surface defects and long imaging times (approximately 8 minutes). In addition, SPGR imaging uses gradient and radiofrequency spoiling to reduce artifacts and achieve near T1 weighting. This reduces the overall signal compared with steady-state techniques. Despite these limitations, 3D-SPGR is considered the standard for morphologic imaging of cartilage.
Both SPGR and GRE techniques produce excellent quality images with high resolution (0.3 × 0.6 × 1.5 mm). The SPGR method suppresses signal from joint fluid, whereas the GRE method accentuates it. Compared with balanced steady-state free precession (SSFP), which is described later in greater detail, these methods are less SNR efficient but also less sensitive to magnetic field inhomogeneity. Therefore, an ideal 3D cartilage imaging sequence that provides an optimal combination of resolution, SNR efficiency, and minimal artifacts has yet to be established. As such, a number of newer techniques have been established to improve cartilage imaging.
NEW MRI METHODS
DESS Imaging
Dual-echo steady-state imaging (DESS) has proved useful for evaluation of cartilage morphology. This technique acquires two gradient echoes separated by a refocusing pulse and then combines both echoes into the image. This results in an image with higher T2* weighting, which has bright cartilage signal and bright synovial fluid.
DEFT Imaging
Driven equilibrium Fourier transform (DEFT) has been used in the past as a method of signal enhancement in spectroscopy. The sequence uses a 90-degree pulse to return magnetization to the z-axis, increasing signal from tissue with long T1 relaxation times such as synovial fluid. Unlike conventional T1- or T2-weighted MRI, the contrast in DEFT is dependent on the ratio of the T1/T2 of a given tissue.
For musculoskeletal imaging, DEFT produces contrast by enhancing the signal from synovial fluid rather than attenuation of cartilage signal as in T2-weighted sequences. This results in bright synovial fluid at short repetition times (TR). At short TR, DEFT shows much greater cartilage to fluid contrast than SPGR, PD-FSE, or T2-weighted FSE.
DEFT imaging has been combined with a 3D echoplanar readout to make it an efficient 3D cartilage imaging technique. In DEFT there is no blurring of high spatial frequencies such as in PD-FSE. Unlike T2-weighted FSE, cartilage signal is preserved owing to the short echo time (TE). A high-resolution 3D dataset of the entire knee using a 512 × 192 matrix, a 14-cm field of view (FOV), and 3-mm slices can be acquired in about 6 minutes. Initial studies of cartilage morphology have been done using DEFT imaging, but this technique has not been conclusively proved superior to 2D approaches. A sequence similar to DEFT that has been used in musculoskeletal imaging is FSE with driven equilibrium pulses, referred to as DRIVE.
Balanced Steady-State Free Precession Imaging
Balanced steady-state free precession (SSFP) MRI is an efficient, high-signal method for obtaining 3D MR images. Depending on the manufacturer of the MRI scanner, this method has also been called True-FISP (Siemens), FIESTA (General Electric), or balanced FFE imaging (Phillips). With recent advances in MR gradient hardware, it is now possible to use balanced SSFP without suffering from the banding or off-resonance artifacts that were previously a problem with this method. However, banding artifacts due to off resonance are still an issue as TR increases, or at 3.0T. Hence, TR is usually kept below 10 ms with these techniques, which limits overall image resolution. Multiple acquisition SSFP can be used to achieve higher resolution at the cost of additional scan time.
Fat Suppression in Balanced SSFP Imaging
Many methods have been proposed to provide fat suppression with SSFP imaging. If the repetition time is sufficiently short and the magnetic field homogeneous, conventional fat suppression or water excitation pulses can be used. Linear combinations of SSFP and fluctuating equilibrium MRI (FEMR) use the frequency difference between fat and water and multiple acquisitions to separate fat and water. Intermittent fat suppression uses transient suppression methods to provide intermittent fat saturation pulses and suppress lipid signal. Iterative decomposition of water and fat with echo asymmetry and least-squares estimation (IDEAL) uses multiple acquisitions to separate fat and water but does not depend on the fat-water frequency difference to constrain the repetition time. Rapid separation of water and fat can be achieved with phase detection. Fat and water separation has also been achieved with phase detection and a radial acquisition method using multiple echoes.
FEMR Imaging
Fluctuating equilibrium MRI (FEMR) is a variant of SSFP that may be useful in imaging cartilage. Similar to DEFT, FEMR and other SSFP-based sequences produce contrast based on the ratio of T1/T2 in tissues. With appropriate choice of flip angle, this results in bright fluid signal while preserving cartilage signal. In scanning the entire knee, FEMR can produce 3D images with a 2-mm slice thickness using a 512 × 256 matrix over a 16-cm FOV in about 2 minutes and 30 seconds. The TR was set at 6.6 ms at 1.5 T, which can be used for fat/water separation with careful shimming to minimize artifacts. An example water image using high-resolution FEMR is shown in Figure 8-3 , compared with a 3D-SPGR image that took almost 9 minutes to acquire.


Linear Combinations of Balanced SSFP and Fat-Suppressed SSFP
Other SSFP approaches may provide more reliable fat suppression at high resolution than FEMR. These methods include linear combination balanced steady-state free precession (LC-SSFP), which uses multiple acquisitions to create fat and water images. Fat-suppressed SSFP uses intermittent fat saturation pulses with preparation pulses that allow transitions in and out of the steady state.
IDEAL SSFP
Another approach to fat/water separation that is relatively insensitive to field variations combines IDEAL with SSFP. Example knee images using this technique are shown in Figure 8-4 . Excellent separation of fat and water is seen, with little off-resonance artifact. This method works at both 1.5T and 3.0T.




Phase-Sensitive SSFP Imaging
Phase-sensitive (PS) SSFP employs an SSFP sequence with the TE restricted to be one half of the TR. The spectral response of the signal with respect to resonance frequency is periodic. The periodicity decreases with decreasing TR, resulting in less field inhomogeneity sensitivity. Voxels are assigned to water or fat to form two separate images. This method is a rapid means of fat water separation using SSFP and does not require additional acquisitions or saturation pulses. One drawback to this approach is partial volume artifact, because pixels are assigned as either fat or water, so high resolution is required. Example images of this method are shown in Figure 8-5 . These images show a 3D, fat-suppressed dataset of an entire knee that can be acquired with 0.625 × 0.625 × 2 mm resolution in about 90 seconds. In a recent limited study, PS-SSFP was sensitive to marrow edema and meniscal tears in a similar manner to FSE imaging.

Vastly Interpolated Projection Reconstruction Imaging
Imaging of the knee with a combination of a 3D radial k-space acquisition and SSFP has several advantages. The 3D radial acquisitions are often undersampled in sparse, high-contrast imaging environments such as contrast-enhanced MR angiography (CE-MRA) to decrease imaging time. Vastly interpolated projection reconstruction (VIPR), first developed for time-resolved CE-MRA, was later adapted for SSFP imaging of the musculoskeletal system. Instead of using the radial trajectory to undersample in musculoskeletal imaging, the radial acquisition allows for a very efficient k-space trajectory that collects two radial lines each TR without wasting time on frequency dephasing and rephasing gradients. One radial line begins at the k-space origin, while the other is acquired along a different return path to the origin, allowing acquisition to occur during nearly the entire TR. The optimal TR needed for the most efficient implementation of linear combinations of SSFP at 1.5T (2.4 ms) can be met while still having time for adequate spatial encoding.
Application of VIPR to the knee provides isotropic 0.5- to 0.7-mm 3D imaging that allows for reformatted images in arbitrary planes. Because this method is based on SSFP, joint fluid is bright, providing excellent contrast for the diagnosis of meniscal tears, ligament injuries, and cartilage damage. Contrast between the cartilage and bone is generated by separating fat and water with linear combinations of SSFP, as shown in Figure 8-6 . Scan time for the isotropic acquisition was only 5 minutes. An alternative single-pass method separates fat and water by exploiting the different phase progression of fat and water spins between the two echoes acquired each TR. At 3T, fat and water separation is achieved by using an alternative fat stopband with a TR of 3.6 ms. Here the multi-echo acquisition allows for the removal of the unwanted passband between the water and fat resonance frequencies at the longer TR.


3D FSE Imaging
Recent advances in parallel imaging technology have enabled 3D FSE imaging with reasonable imaging times. These methods also commonly employ a method of flip angle modulation along the echo train to reduce the effects of blurring. When combined with multi-channel coils, isotropic resolution on the order of 0.6 mm can be achieved in an imaging time of 5 to 8 minutes. This enables one to obtain reformatted images of articular cartilage as well as be able to evaluate ligaments, menisci, and other important joint structures. An example of using 3D FSE imaging for articular cartilage in the knee is shown in Figure 8-7 .


High-Field MRI
High-field MRI may enable the acquisition of morphologic images at spatial resolutions that cannot be achieved in a reasonable scan time at 1.5T. Currently, 3.0T MRI units are available that, theoretically, have twice the signal to noise of 1.5T scanners. In addition, the increased chemical shift allows for shorter fat suppression or water excitation pulses, improving the speed of 3D-SPGR and 3D-GRE scans. IDEAL fat/water separation is also available at 3.0T, with both SPGR and GRE imaging, as shown in Figure 8-8 . Also available are fat, water, and combined images that are corrected for chemical shift. This method could be used to measure subchondral bone thickness. Other fat suppression methods for balanced SSFP imaging, such as FEMR and LC-SSFP, are less applicable to high-field MRI because the shortest TR during which the relative phase of fat and water changes by π is only 1.1 ms. This TR is too short to create any meaningful spatial encoding, and the radiofrequency power deposition would be high.



Sodium MRI
Atoms with an odd number of protons and/or neutrons possess a nuclear spin momentum and therefore exhibit the MR phenomenon. Sodium-23 ( 23 Na) is an example of a nucleus other than hydrogen-1 ( 1 H) that is useful in cartilage imaging. The Larmor frequency of 23 Na is 11.262 MHz/T, compared with 1 H at 42.575 MHz/T. This means that at 1.5T the resonant frequency of 23 Na is 16.9 MHz, whereas it is 63 MHz for 1 H. The concentration of 23 Na in normal human cartilage is about 320 µM, with T2 relaxation times of between 2 and 10 ms. The combination of lower resonant frequency, lower concentration, and shorter T2 relaxation times than 1 H make in-vivo imaging of 23 Na challenging. Sodium imaging requires the use of special transmit and receive coils, as well as relatively long imaging times to achieve adequate signal to noise.
Sodium MRI has shown some promising results in the imaging of articular cartilage. This is based on the ability of sodium imaging to depict regions of proteoglycan depletion. 23 Na atoms are associated with the high fixed-charge density present in proteoglycan sulfate and carboxylate groups. Some spatial variation in 23 Na concentration is present within normal cartilage. Figure 8-9 shows an example of a sodium image through the patellar cartilage of a healthy volunteer done with a twisted-projection technique at 7.0T. High sodium concentration is seen throughout the normal cartilage. In cartilage samples, sodium imaging has been shown to be sensitive to small changes in proteoglycan concentration. This method shows promise in being sensitive to early decreases in proteoglycan concentration in osteoarthritis. It is also possible to do triple quantum-filtered imaging of sodium in cartilage, which may be even more sensitive to early changes.
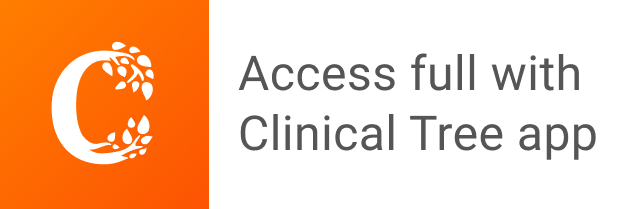