The primary function of articular cartilage is to provide a smooth transfer of force across joints. As such it is useful to consider the cartilage and the underlying subchondral bone as a functional osteochondral unit that must withstand a combination of compressive, tensile, and shear stresses. The stresses are not trivial. For example, it is estimated that with deep knee bending the patellar cartilage undergoes compressive loads that are greater than 6 times body weight. With certain athletic activities such as running, jumping, or weight lifting the forces applied to the joints can reach 10 to 20 times body weight. When considering that human joints are exposed to forces of this magnitude over millions of cycles it is remarkable that normal joint function can be sustained into old age in many individuals. The resiliency of cartilage is due to the highly organized tissue structure. The first portion of this chapter is an overview of osteochondral anatomy as it relates to tissue biomechanics and joint function. The focus in the second half of the chapter is on the role of cartilage structure on the MRI appearance, with particular emphasis on the effect of collagen structure on cartilage T2-weighted signal intensity.
CARTILAGE COMPOSITION
The biomechanical tissue properties of articular cartilage reflect the composition and organization of the extracellular matrix. Articular cartilage is hypocellular and composed of approximately 4% chondrocytes by wet weight. The extracellular matrix is 65% to 85% water, with water content decreasing slightly with depth from the articular surface. The major solid components of the extracellular matrix are type II collagen, which comprises 10% to 20% of the wet weight of cartilage, and large molecules of aggregating proteoglycans, termed aggrecan, that contribute 5% to 10% of the wet weight. Most of the extracellular water is in the solution domain of the aggrecan molecules and is freely exchangeable with synovial fluid; however, a small component is bound in the interfibrillar space of the collagen fibrils.
The content and structure of collagen and proteoglycans in the matrix differ substantially from bone to the articular surface and strongly influence the biomaterial properties of cartilage. The components of type II collagen, tropocollagen molecules, are polymerized into larger collagen fibrils, which, in turn, are organized into a leaflike architecture. As illustrated in Figure 2-1 , the orientation and alignment of the collagen matrix vary with depth from the articular surface as well as regionally within the joint. Collagen fibrils cross the bone/cartilage interface at the tidemark zone and secure cartilage to the subchondral bone. In the deep layer of cartilage near bone, collagen fibrils have a preferential orientation perpendicular to the bone surface. This is frequently termed the radial zone, referring to the radial orientation of the collagen matrix as well as the columnar alignment of chondrocytes observed in this layer. Near the surface, the orientation of the collagen matrix curves tangentially in the transitional zone, becoming parallel to the articular surface in the superficial zone. Initial studies of the transitional zone suggested a decreased level of orientation of collagen fibrils in this layer; however, more recent studies using MRI and cryofracture scanning electron microscopy demonstrate anisotropy in this layer with a preferential orientation oblique to the articular surface. The thickness of the transitional layer increases near the periphery of the articular surface. The superficial layer is covered with a distinct layer of dense collagen fibers termed the lamina splendens. The smooth surface of the lamina splendens, along with the production of unique proteoglycans such as lubricin, surface zone protein, and constituents of synovial fluid greatly reduce friction at the articular surface.

In addition to the zonal variation of the type II collagen matrix, with respect to depth from the articular surface, there are regional differences in composition and organization of collagen within the joint. Regions that are habitually exposed to compressive load, such as the central region of the femoral tibial joint, generally have a thick radial zone and a thinner transitional zone. In addition, habitually loaded regions of cartilage are condensed into thicker fibrils that are spaced at regular intervals. This periodicity is lacking in habitually unloaded cartilage, where collagen fibrils are thinner and have a more random arrangement. Near the periphery of the joint where cartilage experiences primarily shear stress, the transitional zone is thicker and the preferential orientation of the fibers reflects the prevailing direction of shear strain. In addition to regional variation in type II collagen there are also regional differences in expression of nonfibillar collagen proteins responsible for maintaining the organization of the collagen matrix.
Interposed in the meshwork of type II collagen fibrils are large molecules of aggregating proteoglycans consisting of aggrecan. As with collagen and water content, the concentration of proteoglycans also varies within cartilage, with the highest levels found in the middle section and decreasing in concentration near bone and at the articular surface. As illustrated in Figure 2-2 , aggrecan is composed of a central core fiber filament of hyaluronic acid. Through a linking protein the aggrecan monomers are bound to the hyaluronic acid filament. Aggrecan monomers are composed of a central core protein that binds to large, negatively charged glycosaminoglycan molecules consisting of chondroitin sulfate and keratin sulfate. The ionic groups of the chondroitin sulfate and keratin sulfate confer a net negative fixed charge density to the aggrecan in solution. When these molecules are hydrated, the high density of negative charges on the proteoglycans binds sodium and draws water into cartilage through osmotic pressure, causing the proteoglycans to swell. Swelling of proteoglycans is constrained by the surrounding collagen meshwork, producing an interstitial fluid pressure of approximately 9 MPa. This pressurization contributes to the compressive stiffness of cartilage, an essential feature for normal cartilage function.

Also critical to the normal cartilage function is the interface with subchondral bone. The subchondral plate illustrated in Figure 2-3 consists of the tidemark zone representing the junction of calcified and uncalcified cartilage, the zone of calcified cartilage, lamellar subchondral cortical bone, and the underlying trabeculae. Collagen fibers in the radial zone extend through the tidemark zone into calcified cartilage but have not been demonstrated to pass into the underlying cortex. The osteochondral junction represents a potential cleavage plane in response to shear stress. The thickness of the subchondral plate varies with respect to the biomechanical force applied to it and thus will vary depending on joint geometry, loading conditions, and factors such as age, weight, and exercise. The subchondral plate can remodel in response to altered biomechanics that may occur as a result of joint injury and damage to overlying cartilage.

CARTILAGE BIOMECHANICS
In activities of daily living, such as walking, the contact region of one articular surface quickly passes over the opposing surface. During this instant the contact pressure at any one point will rapidly rise in a fraction of a second. When this occurs, cartilage deforms, thereby increasing the local joint congruity and contact area. The intensity of the force imparted onto the articular surface per unit area is termed stress. The deformation of the tissue in response to the applied stress is the tissue strain. When cartilage is loaded, different forms of strain are generated within the tissue. Portions of the tissue are compressed while others are stretched, placing the tissue under tension. In many biologic tissues, such as cartilage, the strain that develops within the tissue in response to the applied stress will vary over time. These tissues are said to demonstrate viscoelastic properties, which in cartilage are a function of the interaction of the three principal components: water, type II collagen matrix, and aggrecan.
Compression
As cartilage is compressed it deforms, producing bulk flow of water through the extracellular matrix. As the cartilage deforms, fluid is exuded into the synovial space. Because of the viscoelastic properties of cartilage the stiffness of the tissue is a function of the rate at which the compressive force is applied. Cartilage is stiffer when the force is applied rapidly and demonstrates less compressive stiffness when the force is applied slowly. The ability of cartilage to resist compression is a result of the ability of the extracellular matrix to limit water permeability. In healthy cartilage, the frictional drag forces of water flow dissipate the vast majority of energy imparted into the tissue during compression. As water movement becomes less restricted, more of the energy is imparted to the collagen and aggrecan matrix, leading to tissue degradation. Resistance to water flow is a function of the high osmolarity, viscosity, and interstitial pressure of the extracellular matrix.
An important function of cartilage is to transfer the compressive force to the underlying bone, which is substantially stiffer. Cartilage demonstrates a depth-dependent difference in compressibility, becoming progressively stiffer toward bone. During physiologic loads most of the tissue deformation occurs in the superficial layer of cartilage, with very little compression occurring in the radial zone near the cartilage/bone interface. The depth-dependent response of cartilage to compressive load is illustrated in Figure 2-4 .

Tension
As the contour of cartilage is perturbed a portion of the tissue is placed under tension. The ability of cartilage to withstand tensile strain is primarily a function of the fibrillar type II collagen meshwork that is anchored to the calcified tidemark zone of cartilage. The hydrated aggrecan contributes to the tensile stiffness of cartilage by buttressing the collagen meshwork.
Shear
Shear stress occurs when a force is applied tangentially to the surface of a material. In the case of cartilage, shear stress occurs primarily at the articular surface as one cartilage surface passes over the other, and at the bone/cartilage interface, where differences in compressive stiffness of these tissues can produce shear strain during high compressive loads.
For normal cartilage the articular surface has a coefficient of friction that is several orders of magnitude less than man-made materials. This is in part due to the smooth surface of the lamina splendens. Specialized molecules in the surface layer of cartilage such as superficial zone protein and lubricin provide important boundary lubrication. In addition, the extrusion of fluid from the cartilage surface as it is transiently compressed provides a fluid gliding layer that reduces shear strain. In the tangential zone the type II collagen fibrils have a preferential alignment along the direction of habitual shear strain within the joint. As shear strain develops in the surface layer of cartilage, this strain is transferred as tensile strain to the underlying collagen meshwork. Excessive tensile strain can produce fractures within the collagen matrix, leading to cartilage fissures along the collagen “leaves” or flap-type tears at the junction of the transitional and radial cartilage zones.
Differences in biomaterial properties of cartilage and bone can lead to high shear strain at the tidemark zone. As illustrated in Figure 2-4 , compressive forces transmitted to the deep layers of cartilage from the articular surface are transferred into shear force as they reach the cartilage/bone interface. This can lead to cleavage of the radial fibers at the tidemark zone, resulting in cartilage delamination. As described previously, the radial collagen fibrils of the radial zone pass through the tidemark, terminating in the zone of calcified cartilage. Because the fibers do not pass into the subchondral cortex there is the potential for cleavage between the calcified cartilage and subchondral bone.
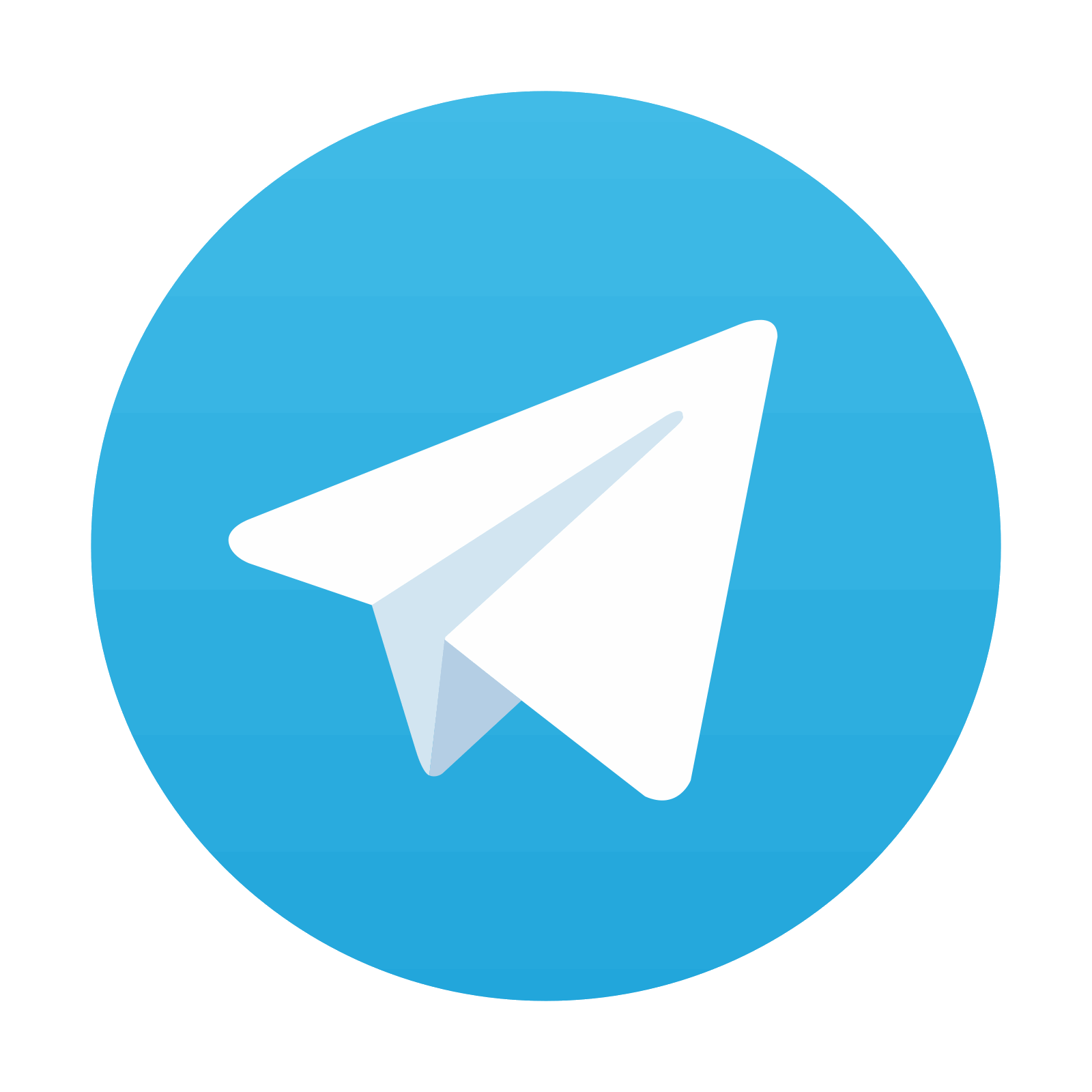
Stay updated, free articles. Join our Telegram channel
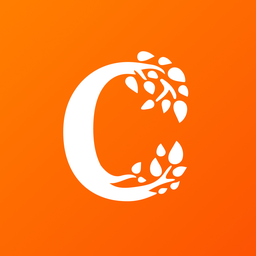
Full access? Get Clinical Tree
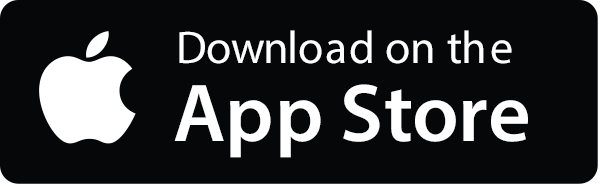
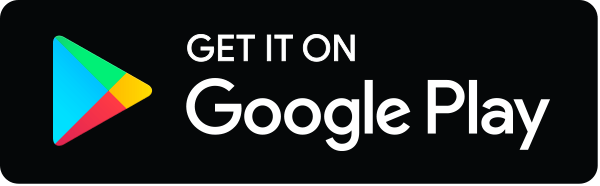