Fig. 11.1
The number of moderate and severe neck injuries in motor vehicle crashes is at historical lows
11.2 Anatomy
The complete spine is a structure composed of 7 cervical, 12 thoracic, 5 lumbar, 5 sacral and 4 coccygeal vertebrae. Each vertebra is composed of a cylindrical vertebral body connected to a series of bony elements collectively referred to as the posterior elements. The posterior elements include the pedicles, lamina, spinous and transverse processes, and the superior and inferior facet joint surfaces. This structure is also known as the neural arch. It provides mechanical protection for the spinal cord and contributes to the stability and kinematics of the vertebral column.
The cervical spine is comprised of seven vertebrae that form eight motion segments between the base of the skull and the first thoracic vertebra, T1 (Fig. 11.2). In the neutral upright posture, the cervical spine has slight curvature with a posterior concavity that is referred to as the normal lordosis. The vertebrae are numbered such that the upper most vertebra is denoted C1, also called the atlas, and the lowermost vertebra is C7. The motion segments are similarly labeled C1 through C8. The C1 motion segment denotes the articulation of the base of the skull with the first cervical vertebra and C8 denotes the articulation of the C7 vertebra with T1. Because this nomenclature scheme gives an identical name to the motion segment superior to the vertebra of the same name, e.g., motion segment C4 is superior to the C4 vertebra, an alternate labeling scheme is often used for clarification. The motion segment and intervertebral disc between two vertebrae can be described with reference to both surrounding vertebrae. For example, the C4 motion segment is also called the C3–C4 motion segment. These vertebrae and motion segments can be structurally grouped morphologically and mechanically into two regions, the upper cervical spine and the lower cervical spine.
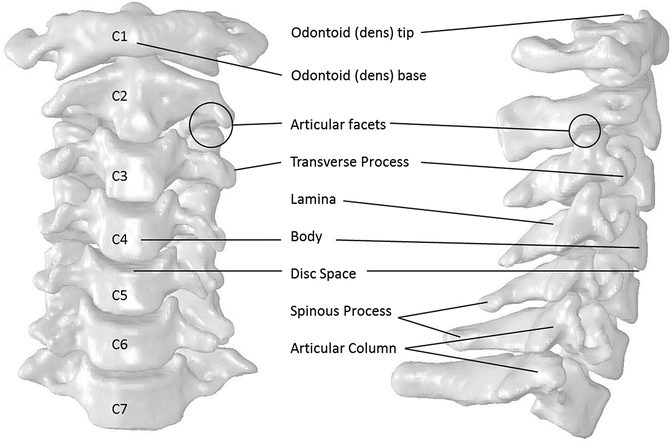
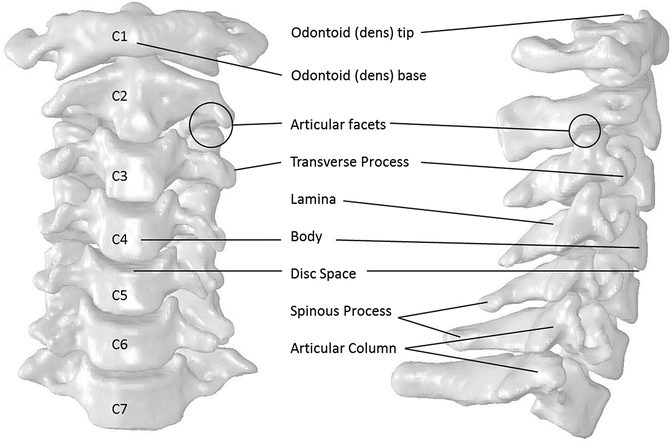
Fig. 11.2
The anatomy of the cervical spine (anatomical reconstruction from the NIH visible human project). The slight curvature that is visible on the right is called the lordosis
The vertebrae of the upper cervical spine (or UCS) are anatomically distinct and consist of three bony elements: the occiput (the base of the skull), the atlas (C1) and the axis (C2) (Fig. 11.3). They produce two joints – the occipito-atlantal joint (C1 or O-C1) and the atlantoaxial joint (C2 or C1–C2). Unlike the other vertebrae, the atlas has no vertebral body. The atlas is a bony ring with enlarged facets on the lateral portions of the ring, and is divided into the anterior and posterior arches. The anterior arch forms a synovial articulation with the second cervical vertebra, the axis, and the posterior arch provides protection for the spinal cord and brainstem. Like the vertebrae in the lower cervical spine, the axis is composed of a vertebral body and a posterior bony arch. But it also has an additional element called the odontoid process (the dens) that is embryologically derived from the fusion to C2 of what would have been the C1 vertebral body. The odontoid process points superiorly from the C2 vertebral body, and its anterior surface articulates with the posterior portion of the anterior arch of the atlas. The lateral portions of the axis contain flattened, enlarged articular facet surfaces and lateral to these surfaces are the transverse processes.
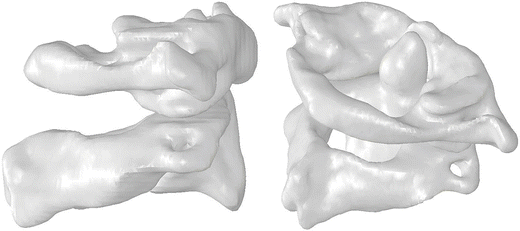
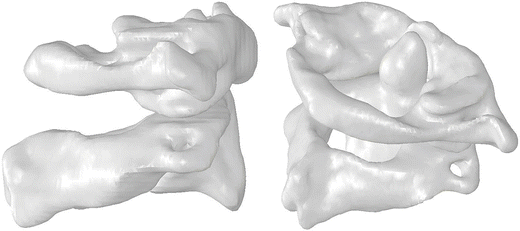
Fig. 11.3
Saggital and oblique views of the upper cervical spine. C1 (the Atlas) is a ring shaped vertebra with no body. C2 (Axis) has a bony process called the odontoid or dens about which C1 can rotate
The O-C1 joint, which allows large flexion-extension rotations, is formed by the superior facets of the atlas and two bony protuberances on the base of the skull called the occipital condyles. The C1–C2 joint, which allows large axial head rotations, is composed of three synovial articulations between the atlas and the axis. The superior facet surfaces of the axis form two synovial articulations with the inferior facet surfaces of the atlas. The third synovial joint is formed by the articulation of the anterior arch of the atlas with the odontoid process of the axis.
The ligamentous structures of the occipitoatlantoaxial complex include continuations of the lower cervical ligaments and an additional set of structures unique to the upper cervical spine. The transverse ligament is a stout horizontal ligament that connects the medial portions of the C1 lateral masses and constrains the odontoid process posteriorly. The transverse ligament is the horizontal portion of the cruciate ligament. The vertical portion of this ligament attaches to the anterior inferior aspect of foramen magnum of the base of the skull and the posterior aspect of the C2 vertebral body. The apical ligament is a midline structure that connects the apex of the odontoid to the base of the skull anterior to the cruciate ligament. The alar ligaments originate on the posterior lateral aspect of the odontoid process and ascend laterally to insert directly to the base of the skull. The posterior longitudinal ligament, which runs the entire length of the spinal column, inserts on the base of the skull posterior to the transverse ligament and is named the tectorial membrane. The anterior longitudinal ligament (ALL) inserts on the base of the skull. The anterior atlantoepistrophical and atlantooccipital ligaments lie posterior to the ALL and connect the C2 vertebral body to the atlas and the atlas to the base of the skull, respectively. The lower cervical flaval ligaments insert on the base of the skull and are denoted the posterior atlanto-occipital membrane. Finally, the nuchal ligament, which is formed by the midline fusion of the paravertebral muscular fascia, inserts on the occipital protuberance, which is small bump on the back of the head just above the neck.
The lower cervical spine (or LCS) contains vertebrae C3 through C7 and motion segments C3 through C8. The vertebrae, which increase in absolute size from superior to inferior, are geometrically similar with a roughly cylindrical vertebral body and posterior bony arch. The vertebral bodies are connected to one another by a fibrocartilaginous intervertebral disc. The disc is composed of a central fluid-like nucleus pulposus bounded by a laminar set of spirally wound fibrous sheets called the annulus fibrosus. The anterior surfaces of the vertebral bodies are connected from the sacrum to the base of skull by the ALL (Fig. 11.4). Similarly, the posterior longitudinal ligament (PLL) connects the posterior surface of the vertebral bodies and forms the anterior surface of the spinal canal. The pedicles run posterolaterally from the posterior of the vertebral bodies and terminate in the articular column (or pars interarticularis). The articular column denotes a bony region bounded superiorly by the superior facet surface, inferiorly by the inferior facet surface, laterally by the transverse process, and medially by the spinal canal. Lateral to these elements are the transverse processes, which contain the vertebral artery, the major blood supply for the brainstem and the posterior portions of the brain. This artery is contained in the foramen transversarium of the transverse process. The lamina project posteromedially from the pars interarticularis and meet at the midline. The lamina form the posterior lateral surface of the spinal canal. Protruding from the midline fusion of the two lamina on each vertebra is the spinous process. The spinous processes are the palpable protuberances on the dorsal surface of the neck and back.
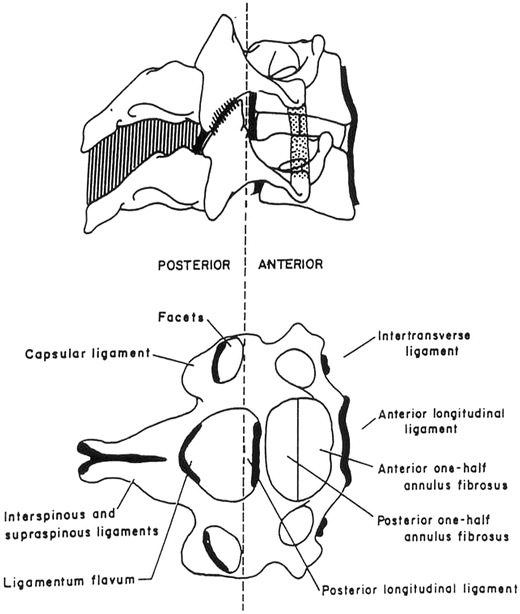
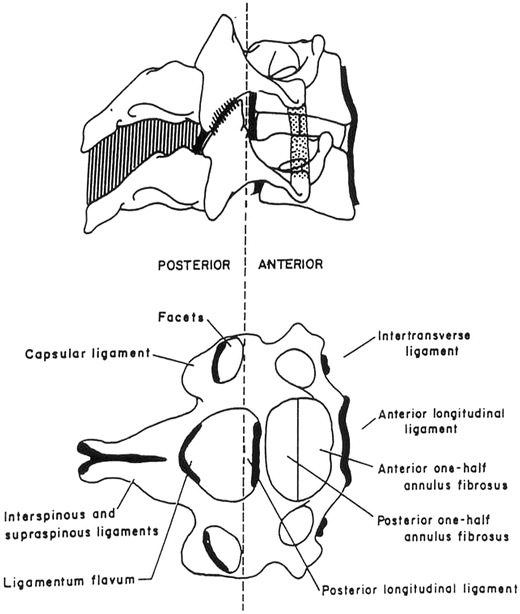
Fig. 11.4
A schematic of the cervical spine ligaments
The interspinous and supraspinous ligaments connect the spinous processes of adjacent vertebra. The flaval, yellow, ligaments similarly connect adjacent lamina from the sacrum to the base of the skull. The facet joints are formed by the inferior facet surface of the superior vertebra and the superior facet surface of the inferior vertebra. These are synovial joints, which are wrapped in a capsular ligament with paraspinal muscular insertions. The spinal canal of the vertebrae is the location of the spinal cord. Nerve roots exit between adjacent vertebrae through the intervertebral foramen. This foramen is composed of the inferior vertebral notch of the superior vertebra and the superior vertebral notch of the inferior vertebra.
11.3 Fractures and Dislocations
In this section, we review the more common fracture patterns and their association with spinal cord injuries (SCIs). The purpose is to present the clinical picture, without regard to the mechanism of injury, so that the reader has some context for the relationship between cervical spinal fracture (AIS 3) and spinal cord injury (AIS 5+). Understanding the dynamics of fracture, the stresses created on the spinal cord during fracture, and the potential for SCI is an exceptionally complex biomechanical problem. Making a robust prediction of specific fractures is well outside the scope of our current understanding and computational abilities. Fortunately, however, it is possible to mechanistically understand the probability of SCI by examining the extent to which the spinal cord is impinged during a typical fracture and to validate that understanding against clinical studies that provide SCI frequency for particular fractures.
Broadly speaking, lower cervical spinal (LCS) cord injuries are caused by burst fractures, dislocations, and fracture-dislocations. Cord injuries are much less common with simple fractures (i.e. vertebral compression fractures and other minor fractures). Spinal cord injuries are also associated with fractures that are unique to the upper cervical spine (UCS), so these are discussed separately.
11.3.1 Lower Cervical Spine
Burst fractures typically include both the anterior and posterior cortex of the vertebral body (Fig. 11.5). They occur in the lower cervical spine at all vertebral levels from C3 to T1 and are responsible for 30 % of spinal cord injuries [28]. These lesions may consist of destruction of the cancellous bony centrum with loss of disk height and/or vertebral end-plate and multi-part fractures of the vertebral body. One variation of burst fractures is a midsagittal cleavage fracture. The most common sites of these fractures in the lower cervical spine are C4, C5, and C6 [25]. Because the vertebrae are a closed bony ring, complete fracture through the anterior and posterior cortex of the vertebral body is often accompanied by fractures to the lamina, and disruption of the facet joints. They are grossly unstable injuries and bony fragments, often trapezoidal in shape, are displaced posteriorly and impinge on the spinal cord. The risk of spinal cord injury from these fractures is very high in the reported literature (deficit on admission >90 %, complete lesions 40–75 %), and tends to increase with the severity of the fracture [29].
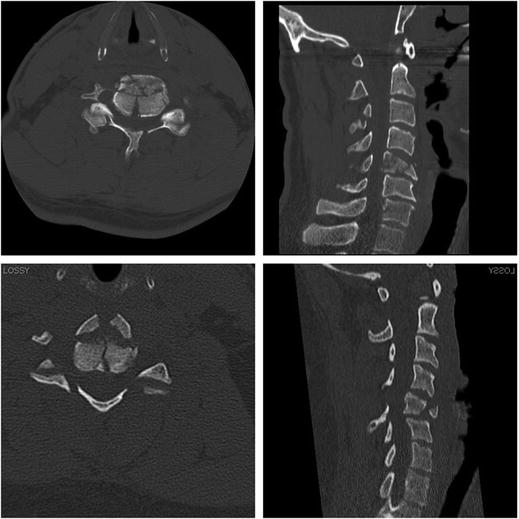
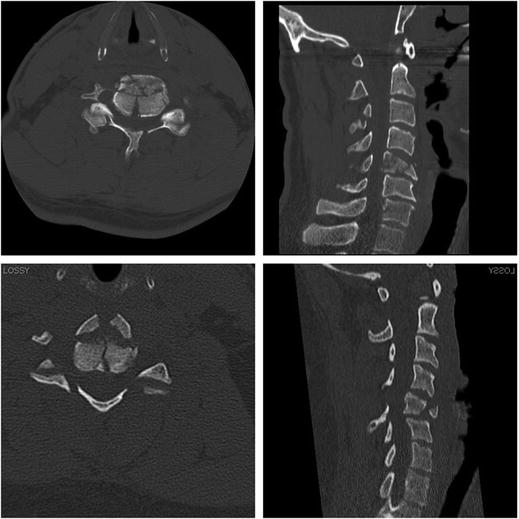
Fig. 11.5
Burst fractures of C5 from a mountain bike crash (top) and a shallow pool dive (bottom). Note the retropulsion of bone fragments into the spinal canal and the anterior “tear drop” fragment on the sagittal reconstructions (right)
A cervical spine dislocation typically refers to an injury in which the superior vertebral body is displaced (subluxed) relative to its subjacent vertebra. This displacement, which is most often in the anterior direction, results in disruption and frequently dislocation of the facet joints with subsequent locking of the surfaces in a tooth-to-tooth fashion secondary to muscle spasm (Fig. 11.6). If both facets are dislocated, it is called a bilateral facet dislocation (or BFD). The lesion occurs at all levels of the lower cervical spine, but predominates between C5 and C7 [30–32]. A pure dislocation without any fracture is relatively unusual and accounts for only 5 % of spinal cord injuries. BFDs are more commonly associated with fractures of the facets and often of the superior-anterior lip of the inferior body, or the anterior-inferior lip of the superior body. This injury results in greater than 50 % anterolisthesis and a significant reduction in the anteroposterior diameter of the neural canal and is therefore usually associated with spinal cord injury. Braakman and Vinken report permanent, partial, or complete spinal cord injury in 27 of 35 patients with bilateral dislocations [33]. Other studies have found that permanent quadriplegia occurs in over 50 % of these injuries [31, 33, 34]. Bohlman found that over one half of patients with tetraparesis had sustained some form of bilateral facet fracture dislocation.
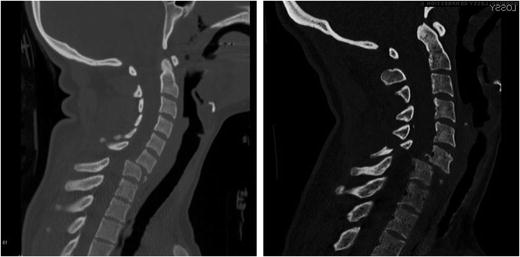
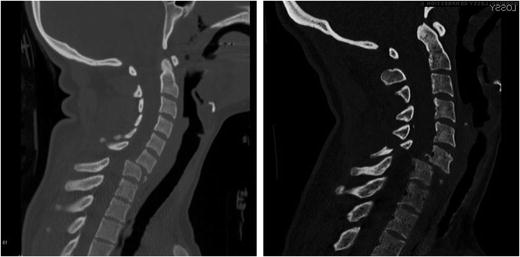
Fig. 11.6
Bilateral facet dislocations of C6–C7 from rollover crashes. A “teardrop” fragment is observed on the left. On the right, there is an associated lamina fracture of C6
Unilateral facet dislocation (UFD) refers to the anterosuperior displacement of only one of the two facets over the facet of the inferior vertebra with subsequent locking in a tooth-to-tooth fashion. The most common sites of this injury are the C5, C6, and C7 motion segments [33]. Clinically, the lesion is frequently asymptomatic, or associated with radicular pain on the side of the injury (25 of 39 patients). Spinal cord injury is relatively un-common in this type of injury [33, 35].
Fracture dislocations are often characterized by comminution of vertebral bodies and gross displacements of the vertebrae (Fig. 11.7). The injured vertebrae may not be contiguous and concurrent injuries to the upper cervical spine may be seen. A typical clinical presentation might involve displaced C6–C7 burst fracture with posterior element fracture and concomitant fracture of C2.
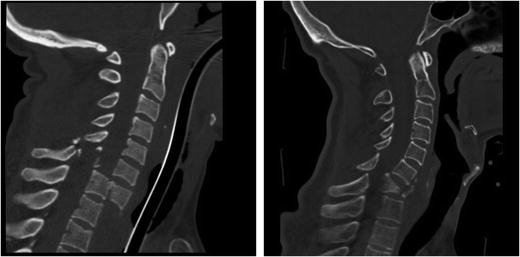
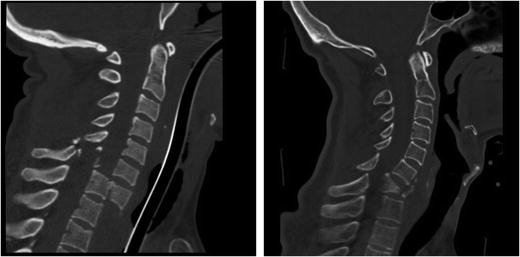
Fig. 11.7
(Left) A severe fracture dislocation of C7-T1 from a rollover crash with full body antero-lithesis and downward translation of the C7 body. (Right) A fracture dislocation of C6–C7 with a burst fracture of the C7 vertebral body from a lacrosse accident
11.3.2 Upper Cervical Spine
Because of its unique anatomy and function, the upper cervical spine fractures in idiosyncratic ways. The clinical literature indicates that many of these injuries do not have neurological involvement, but this reflects a clinical epidemiologic bias. Because damage to the spinal cord at this level is rarely survived, upper cervical spine fractures may be under-represented in the clinical literature because the victims are not transported to emergency rooms [36].
One of the more frequently described injuries to the upper cervical spine is a multipart fracture of the atlas (Fig. 11.8). While these fractures are frequently described as Jefferson fractures, the Jefferson fracture properly refers to a particular four part fracture of the atlas [37]. Fatalities and instability from this group of injuries are common [38, 39].
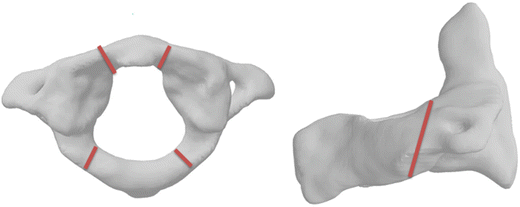
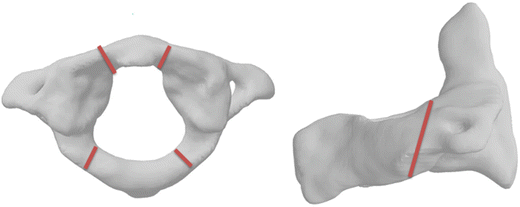
Fig. 11.8
Schematic of the classic four-part Jefferson fracture (left) and the Hangman’s fracture (right)
Traumatic spondylolisthesis of the axis, or Hangman’s Fracture, describes a fracture of the elongated pars interarticularis of the posterior arch of the second cervical vertebra (Fig. 11.8). It has been historically attributed to hyperextension and distraction (tension and rearward rotation of the head), which can result from blows to the face and chin or as its name would suggest, from judicial hanging [2, 38, 40–42]. Rupture of the C2–C3 intervertebral disc accompanies the pars fracture and creates dramatic instability in the more serious forms of the injury. Automobile crashes have replaced hangings as the most common cause of these often fatal injuries [43].
Another important class of C2 injury is odontoid fracture (Fig. 11.9). These injuries are frequently mechanically and clinically unstable and are of considerable importance because of the potential for lethal spinal cord impingement, and the technical difficulty associated with surgical stabilization of the injury. They have been classified clinically by Anderson and D’Alonzo into three groups or types: Type I, avulsion fractures of the superior-most tip of the odontoid; Type II, fractures through the body of the odontoid; and Type III, fractures through the base of the odontoid that extend into the axis vertebral body [44]. Clinically, Type I injuries are stable, and Type III injuries tend to heal with appropriate immobilization. Type II injuries, however, have a high incidence of nonunion, and surgical repair is common. Odontoid fractures are biomechanically important because most of the strongest ligaments attaching the head to the neck insert or originate on the dens. Therefore, these fractures can lead to atlanto-axial (C1–C2) dislocation. An atlantoaxial dislocation can also occur due to transverse ligament rupture; however, a traumatic etiology for this problem is controversial as the transverse ligament is thought to be stronger than the odontoid process [45]. Experimental work by Fielding et al., based on shear loads applied directly to the atlas, suggests that the transverse ligament can fail in postero-anterior shear loading prior to odontoid fracture, indicating that a traumatic transverse ligament injury is possible [46]. A traumatic tearing of the transverse ligament of the axis does occur in patients with preexisting soft tissue laxity or collagen vascular disease (e.g., Down’s Syndrome or rheumatoid arthritis). In these patients the trauma producing the lesion is minor and the injury is described as a spontaneous atlantoaxial dislocation.
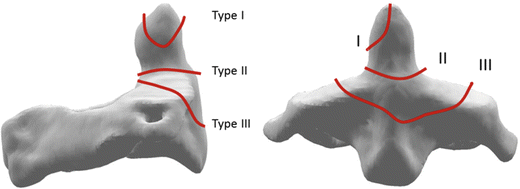
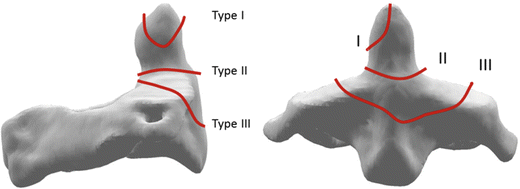
Fig. 11.9
Schematic of the three classes of odontoid (or dens) fracture (Adapted from Anderson and D’Alonzo)
Montane et al. report on four cases of occipitoatlantal dislocation resulting from rapid decelerations in motor vehicle accidents in which the victims survived past the immediate event [47]. The prevalence of this type of injury is debated. Because of the nature of the injury and the method of dissection at autopsy, occipitoatlantal dislocations are frequently undetected and were, therefore, thought to be uncommon. However, according to Buckolz and Burkhead the lesion is a common cause of death in motor vehicle accidents where it occurred in 9 of 26 automotive fatalities with cervical spine injury, making it the most common cervical injury in their study of 112 total automotive fatalities [48].
Another noteworthy upper cervical spine injury is the basilar skull fracture. These are very serious and often fatal injuries that came to attention in the last decade with some high profile fatalities of race car drivers. Reviewed in detail by Myers and Nightingale, basilar skull fractures (BSFs) can occur from a variety of mechanisms, but when the loads on the skull base arise from compression or tension of the cervical spine, the fractures have distinctive characteristics, including the “ring” fracture [49]. These injuries are often immediately fatal owing to rupture of the main blood vessels of the head, and direct injury to brain and brain stem. Clinical presentation can include significant craniofacial bleeding, or bruising around the eyes and ears.
11.3.3 Other Fractures
Clay Shoveler’s fracture is an isolated fracture of the spinous process [42]. It is named for its historical occurrence in people while shoveling clay; the clay sticks to the shovel resulting in a whiplash motion of the head with avulsion of the spinous process. Clinically, the lesion is of little significance, as it is associated with neither neurologic deficit nor spinal instability.
Teardrop, or limbus, fractures refer to the bony avulsion of either the antero-inferior or anterosuperior portion of the vertebral body in the lower cervical spine. These fragments were designated as teardrop by Schneider and Kahn because of their water droplet appearance in the lateral view on X-ray, and because of the strong emotional component frequently associated with such lesions. Because the overall structural integrity of the cervical spine varies considerably with this presentation, a simple relationship between teardrop fractures, spinal stability, and the risk of spinal cord injury does not exist [50]. However, the risk for spinal cord injury due to retropulsed bony fragments associated with the teardrop fracture must always be considered when the teardrop fracture is seen as part of a burst fracture. There has beenextensive discussion of the etiology of teardrop fractures over the years based largely, but not exclusively, on subjective evaluation of patient injury data [41, 42, 50, 51, 52, 53, 54]. Review of the literature reveals a wide variety of mechanisms through which teardrop fragments may be formed and suggests that the size and geometry of the fragment depends as much on host factors, (e.g., local bone strength, ligamentous strength, and the presence of degeneration) as on the type of applied loading. Thus, generalizations about the injury based solely on the presence of a teardrop fracture are not appropriate. The clinical importance of these lesions stems from the recognition of their association with other structural injuries to the spine, and the associated instability and neurologic injury that they can produce. It should be noted that the teardrop fracture was designated as such in an era of plain radiographs, which were not were not able to capture the vertical body fracture planes with the fidelity seen on modern CT and MRI [28]. Therefore, the clinical implications of the teardrop fragment may not be as important in a modern radiological setting.
11.3.4 Clinical Classification
Because our knowledge of cervical spine trauma has evolved from a wide variety of institutions and sources, several authors have attempted to classify the injuries so that there can be agreement on what investigators are referring to when describing a particular injury. These include Allen et al., Babcock, Dolen, Harris et al., Melvin et al., Moffatt et al., Myers and Winkelstein, Portnoy et al., and White and Panjabi [32, 38, 42, 51, 55, 56, 57, 58]. The bases for these classifications has included retrospective reviews of patient data, whole and segmental cadaveric experimentation, and retrospective evaluations of various known injury environments [14, 25, 36, 55]. While most of the above classification schemes are very thoughtful, they have been necessarily speculative and often based on anecdotal or inferential evidence. Injury classifications are further complicated by the terminology used to determine the mechanism. The global motion of the head relative to the torso is often different from, and mistaken for, the motions of the cervical spine at the injured level. For example, the observation of a flexion motion of the head relative to the torso may actually be concurrent with local (i.e. a single motion segment) extension in the cervical spine and may produce an extension injury. Further, experimental studies have shown that small changes of the impact location or the initial position of the head (less than 1.0 cm) can change the injury from compression-flexion to compression-extension in cadaveric studies [59, 60]. In addition, the observed motions of the head may occur after the injury has occurred and thus reflect the motions of an unstable head and spine rather than the true injury mechanism [61].
Understanding whether the classifications are based on global motions of the head (as in [42]), or local deformations of the motion segment [55] is key to an understanding the validity of the injury classification. But all too often in both the clinic and in litigation, the assumption is that the classification refers to motions of the head, not the spine at the site of injury.
11.4 Experimental Studies
Experimental investigations using whole cadavers, whole cervical spines, and cervical spine segments have been invaluable in developing kinematic relationships as well as suggesting injury mechanisms. The primary limitation on such research is the paucity of cadaveric tissue for the study of injury and the lack of active musculature. This lack of tissue restricts the size of most studies and has been, perhaps, the greatest limitation on advancing our understanding of biomechanics. Despite these limitations, the neck injury literature includes far more studies than we can reasonably summarize in this chapter. This section reviews the studies that have had the most impact in shaping our current understanding of cervical spine injury mechanisms. The reader is referred to Myers and Winkelstein for a more comprehensive review of the literature [58].
11.4.1 Compression
Most cervical spine injuries occur when the neck is forced to manage the kinetic energy of the moving torso. This situation is best exemplified by diving injuries, but it also occurs in other athletic/recreational activities as well as in motor vehicle crashes and household accidents. For this reason, much of the research on neck injury mechanisms has been focused on compression. There are a number of experimental studies on the compressive responses of cervical spine segments, but they are of limited utility in understanding injury because of the complex dynamics associated with compressive impact. For this reason, we will limit our review of compression to whole cadaver tests and whole cervical spine tests.
11.4.1.1 Whole Cadavers
Culver et al. performed 11 superior-inferior head impacts of unembalmed cadavers using a padded impactor [62]. No fractures were observed at the peak head forces below 5.7 kN. Mean head impact force producing cervical injury was 7.58 ± 0.94 kN. Most of the injuries produced were in the posterior elements, which the authors attributed to “compressive arching”. These authors were among the first to suggest that neck position, particularly the degree of initial lordosis, influenced the injury mechanism.
Hodgson and Thomas performed a series of quasi-static and impact tests using embalmed cadavers, with one side of the spine exposed to allow for high-speed imaging [20]. The authors concluded that increased constraint of the head in the padded impact surface increased the likelihood of injury to the neck. They also concluded that compression from crown impacts produces exaggerated regional cervical spine flexion and increased the likelihood of fracture or dislocation between C4 and C7. It was one of the first studies to note that global compression of the head and neck could produce local flexion and concomitant shear and described the complex neck dynamics that can lead to a wide variety of compressive injuries to a number of cervical levels.
Nusholtz et al. performed impacts to the head vertex of 12 unembalmed cadavers with a guided 56 kg impactor [63]. Compressive extension cervical injury occurred with the necks buckling in extension or flexion. Head impact loads ranged from 1.8 to 11.1 kN. Nusholtz et al. also performed free-fall drop tests using eight unembalmed cadavers [60]. Drop heights that varied from 0.8 to 1.8 m produced extensive and varied cervical and thoracic fractures and dislocations. Head impact forces ranged from 3.2 to 10.8 kN in these tests. Nusholtz et al. drew a variety of conclusions from these cadaver tests that still remain quite valid. (1) The initial orientation of the spine is a critical factor in influencing the type of dynamic response and injuries produced. (2) Descriptive motion of the head relative to the torso is not a good indicator of neck damage. (3) Energy-absorbing materials are effective methods for reducing peak impact force but do not necessarily reduce the amount of energy transferred to the head, neck, and torso or the damage produced in a cadaver model.
Yoganandan et al. conducted a study to evaluate the effect of head restraints on spinal injuries with vertical impact [64]. Sixteen human male cadavers were suspended head down and dropped vertically from heights of 0.9 to 1.5 m. In 8 of the 16 specimens, the head was restrained to simulate the effect of muscle tone, producing preflexion of the cervical spine in its initial position. Head-impact forces ranged from 3.0 to 7.1 kN in the unrestrained and from 9.8 to 14.7 kN in the restrained specimens. On average, the peak load in the C5 or C6 vertebral body was 70 % lower than the peak head load. Cervical vertebral body damage was observed most commonly when the cadavers remained in contact with the impacting surface without substantial rotation or rebound. This caused the neck to be the major element in stopping the torso motion.
One important factor, not well controlled in all of these whole cadaver impact studies, is the degree of head constraint. When the head is free to rotate on the occipital condyles, bending of the neck occurs with minimal compression. When head rotation is constrained, bending of the neck is restricted and the system becomes much stiffer. The factors that influence head motion are the stiffness and conformability of the impacting surface and the cadaver head. Another factor that cannot be controlled in whole cadaver experiments is the extent to which failure progresses. A certain amount of kinetic energy is available in these tests and failures progress until this energy is dissipated. Finally, it is technically difficult to record the internal forces without violating the integrity of the structure, and because the dynamics are complex, the head impact loads are very different from the cervical spine loads [64, 65]. It is these factors, as well as lack of muscle action, that makes it difficult to determine injury tolerance and mechanisms from whole cadaver tests.
11.4.1.2 Whole Cervical Spines
Experiments on whole cervical spines offer the advantage of measuring the cervical spine loads in situ while preserving the geometry and biomechanics. For this reason they have been the most important experiments for establishing cervical spine injury kinetics and tolerance. One of the first such studies is from Bauze and Ardran, [66]. In 1978, Bauze and Ardran recognized that the biomechanics of the bilateral facet dislocation (BFD) were not well understood and undertook the first systematic approach to producing the injury. This was a very significant study, the implications of which would not become clear until decades later. They were able to produce bilateral facet dislocations in 6 of 14 cadaver cervical spines using an apparatus that applied compression while restricting head rotation. They were the first to describe the resulting buckling deformations and hypothesized that it occurred during compressive impacts. The primary limitations of the study were that it was static, and that a non-physiologic stress riser existed at the point of dislocation to control lower cervical bending. In addition, the loads were applied in discrete steps, and only the maximum compressive load for all specimens was reported (1,422 N).
Myers et al. tested 18 cadaver spines as beam-columns in compression using three different mechanical end conditions for the head: fully constrained, rotationally constrained, and unconstrained [67]. Somewhat to the surprise of the authors, the neck could not be broken in the unconstrained mode, which produced a large amount of flexion bending (>90°) with relatively little compressive force (<300 N). Bilateral facet dislocations could be consistently produced in the lower-cervical spine with a rotationally constrained head end condition. Bilateral facet dislocations occurred with an average of 1,720 N of axial force and 2.9 cm of compressive displacement. The study also examined the fully constrained end condition and found it to be substantially stiffer than the other two. In this mode of loading, wedge and compression fractures were produced in the mid-cervical spine. The average axial force and displacements at failure were 4,810 N and 1.4 cm respectively. Increasing the constraints on head motion resulted in a stiffer response and a greater axial load to failure, but there was no statistically significant difference in the energy to failure between the fully constrained specimens and the rotationally constrained specimens. This suggests that the mode of failure shifted from primarily compressive, to a combined loading (compression-flexion) with significant internal bending.
Yoganandan et al. were the first to examine the dynamics of isolated whole cervical spines in compressive impact [68]. They quantified the resulting complex kinematics and suggested that buckling instability may play a role. They also were the first to observe the decoupling between the head response and the cervical spine response.
Pintar et al., studied the responses of whole head and neck preparations to compressive impacts [69]. In these experiments, the necks were straightened (the lordosis was removed) with between 15 and 30° of head flexion and the crown of the head was impacted with a hydraulic ram. The primary variable was the eccentricity of the occipital condyles over the T1 vertebra, which varied from 0.5 cm posteriorly to 2.5 cm anteriorly. They found that compressive injuries in the mid-cervical column were most likely to occur when the condyles were aligned vertically within 1 cm of T1. However, there was no single biomechanical metric that was predictive of injury. They produced a striking variety of injury patterns and concluded that “for essentially the same loading vector, (crown impact to the head) a variety of different injury mechanisms can be produced depending on the configuration of the spine prior to loading. This implies that without sufficient external data (e.g. scalp laceration) an assumed mechanism of injury from retrospective evaluation of radiographs may not be correct.”
Nightingale et al. studied a variety of head constraints and impact angles in compressive drop tests on cadavers [61, 65, 70]. Compressive failure loads were 1.06 ± 0.27 kN for females with a mean age of 65, and 2.24 ± 0.57 kN for males with a mean age of 62. This difference was statistically significant and indicates that sex should be controlled for in biomechanical testing. The injuries produced included nearly the full spectrum of clinically relevant fractures: burst fractures, Hangman’s fractures, odontoid fractures, bilateral facet dislocations, posterior element fractures and anterior avulsion fractures. The authors also observed first and second order buckling of the cervical spine, which gave rise to complex kinematics that can explain mid-cervical fracture patterns (Fig. 11.10). Another significant finding was the speed with which the fractures occurred – in rigid impacts, the spine failed within 8 ms, and in padded impacts, less than 25 ms. Head rebound was another kinetic observation, leading to the conclusion that impact surface should absorb as much of the kinetic energy of the head as possible so as not to return it to the cervical spine (Fig. 11.11). The study had been designed with the thought that angled impacts (contacts fore or aft of the head vertex) would allow the head and neck to rotate and escape the path of the following torso, but because of the head inertia this frequently did not occur and the cervical column fractured.
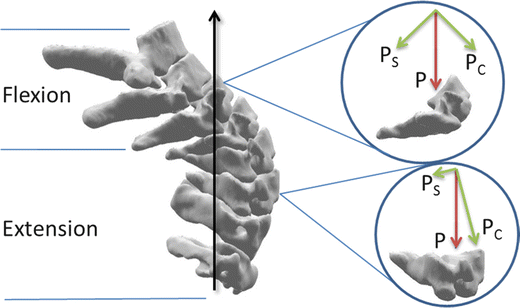
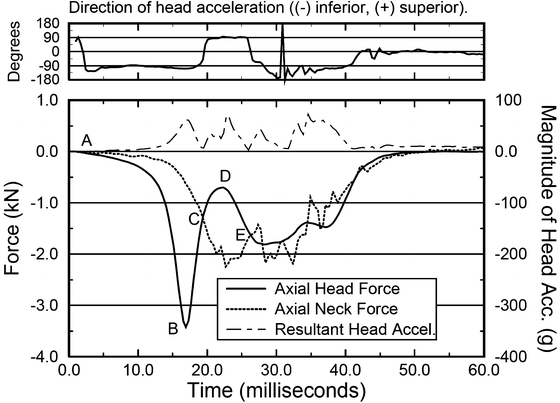
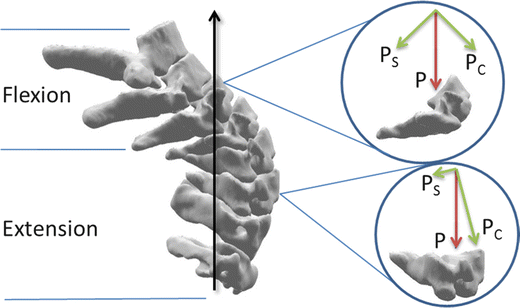
Fig. 11.10
Compressive buckling leads to multiple possible injury mechanisms. C1 through C4 are primarily in compression with extension. C5 through C7 are undergoing shear (s), compression (c) and flexion. Which vertebra fails will depend on timing and subject specific vertebral strengths and geometries
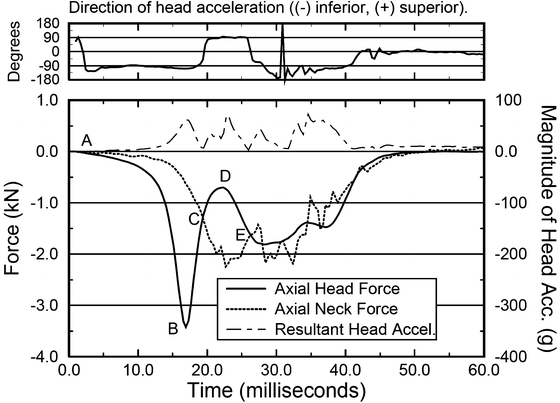
Fig. 11.11
In accordance with Newton’s second law, the difference between the neck force and the head force is M*A of the head. At B the peak head force is always greater than the peak neck force as the head is stopped by the impact surface. The direction of the head acceleration reverses at C as the head rebounds towards the neck. The difference between the curves from C to E is the portion of the neck load due to head rebound
Toomey et al., performed drop tests very similar to those on Nightingale et al., except that they were designed to apply combined compression and lateral bending [71]. It was thought that this configuration might be more relevant to rollover injuries. The fracture loads and timing were not affected by the lateral orientation, nor was the expression of the first order-buckling mode. The authors concluded that the asymmetric loading produces similar kinetics and injury patterns to sagittal plane loading.
More recently, Saari et al., conducted impact tests on whole human cervical spines with high-speed cine-radiography [72]. The injuries produced were primarily compressive-extension fractures of the cervical spine, with multiple “teardrop” avulsions of the anterior vertebral body, all from extension. As with prior impact studies, very little head motion was observed at the time of injury. One specimen had a C1–C2 fracture dislocation with C1 anterior ring fracture and odontoid fracture, which was observed to spontaneously reduce on the cine-radiography. Maximum cord compression occurred between 8 and 15 ms after impact, which supports the notion that cord injury occurs rapidly following head impact.
Ivancic et al., subjected five cadaver spine specimens attached to a Hybrid III dummy head to high-energy horizontal impacts to simulate athletic neck injuries [73]. Catastrophic neck injuries were produced at many levels of the cervical spine. Using the ATD load cell inserted into the occipitocervical junction, the authors observed similar forces and times indicating that inertial forces of the individual vertebrae are small relative to the reactions in the neck and validating the use of T1 load cells as a means to study the neck without violating the cranio-cervical junction.
11.4.1.3 Discussion
The human neck is fairly strong in compression, but it is also very stiff in that direction and not nearly strong enough to manage the kinetic energy of the torso. Head first impacts from low drop heights reliably produce a variety of cervical spine fractures which arise from various buckling modes in the cervical spine [61, 64, 65, 67, 68, 69, 70, 74, 75].
Differences in axial load to failure have been shown to depend on the injury produced and, therefore, on the degree of constraint imposed by the contact surface. From a tolerance standpoint, these injuries should be evaluated separately. As an example, cadaver experiments by Maiman et al., Myers et al., and Pintar et al. show that compression-flexion and compression-extension injuries occur with smaller axial loads than pure compressive injuries [67, 76, 77]. Bilateral facet dislocations reported by Myers occurred at 1,720 ± 1,230 N, and flexion injuries reported by Maiman occurred at approximately 2,000 N. In contrast, compression injuries reported in these studies occurred at 4,810 ± 1,290 N and 5,970 ± 1,049 N, respectively. Given the importance of the musculature in resisting flexion and extension in the lower cervical spine, the above values should be considered as a lower bound for human tolerance in compression-flexion and compression-extension. In contrast, the compressive loads associated with pure compression injuries are a reasonable estimate of cervical compressive tolerance.
Much of the scatter in the existing compressive tolerance data for the cervical spine is due to differences in experimental technique. Since neck responses are very sensitive to end conditions, inertia, and orientations, it is important to use data from studies that employ similar experimental setups. A synthesis of data that meet the inclusion criteria for a dynamic cervical spine tolerance are presented in Nightingale et al. [70]. The results of studies from two institutions were combined to formulate a tolerance for males and females. Averaging the means of the two studies resulted in the following tolerance values: 1.68 kN for females, and 3.03 kN for males. The tolerance values were scaled to account for the age of the cadaver specimens and the authors suggest a cervical spine tolerance for the young human male in the range of 3.64–3.94 kN. The tolerance is lower for a neutrally positioned spine and higher for a straightened spine; however, these are a reasonable first-order representation of the mean resultant force required for a catastrophic cervical spine injury in any given impact situation. The threshold velocity required to produce catastrophic neck injuries is often quoted to be 3 m/s, but catastrophic injuries can occur at even lower velocities during those circumstances in which the neck geometry and initial torso velocity maximize injury potential (i.e. a forward of vertex impact with a straightened spine and the torso velocity perpendicular to the impact surface).
A number of studies have reported on the total compressive displacements of the cervical spine at the time of injury and this metric has been suggested as a measure of tolerance [69]. Compressive impact tests by Pintar et al. with straightened spines resulted in injuries at a mean compressive displacement of 18 ± 3 mm. Myers et al. reported a mean of 14 ± 4 mm in neutrally positioned (lordotic) spines [67]. Displacements can also be estimated from the Nightingale et al., 1997 from the product of injury time and impact velocity for rigid impacts. This results in displacements of 13 ± 7 mm.
Both the neutrally positioned and the straightened cervical spines are stiff structures. As indicated above, the cervical spine generates a great deal of load very quickly and without much compressive displacement. Pintar et al. (straightened spine) produced neck fracture between 1 and 7 ms after impact. Nightingale et al. (lordotic spines) produced injury at an average of 5 ms for rigid impacts and 18 milliseconds for padded impacts. It appears that a burst fracture is more likely in the straight configuration than in the lordotic configuration. A bilateral facet dislocation is more likely in the lordotic configuration than in the straightened. But either injury can happen in either configuration depending on the initial positions, the rate of loading, and chance. The complex deformations resulting from cervical spine buckling explain, in part, why the complete spectrum of cervical spine injuries can be produced in what appear to be very similar compressive impacts [78].
Because cervical spine fracture happens so quickly, there is not enough time for much head motion to occur before the neck breaks. Head rotations greater than 20° have been shown to occur well after neck injury (as much as 80 ms after) and maximum head rotations occur at least 150 ms after injury [61]. In addition, the direction of head rotation (flexion or extension) is not correlated with the neck injury mechanism. The reason for this is that the inertia of head resists changes in linear and angular momentum. This means that it takes both force and time to change the direction and rotation of the head – much more time, it turns out, than it takes to break the neck.
The mechanism of the bilateral facet dislocation (BFD) was long described with biomechanically ambiguous phrases like “forced” flexion or “hyperflexion” and was thought to occur as a result of motion of the head that forces the chin towards the chest. This “hyperflexion” hypothesis was satisfying to lay intuition and became dogmatic in the orthopedic literature [38, 42, 52, 79]. But as early as 1960, difficulties were reported in producing this “flexion” injury in experiments on cadavers [80, 81]. In 1978, Bauze and Ardran were able to produce the injury using an apparatus that applied compression while restricting head rotation [66]. They called the buckling deformation “ducking” and hypothesized that it occurred during compressive impacts. In 1980, Hodgson and Thomas demonstrated these ducking modes of deformation and concluded that compression from crown impacts produces exaggerated regional cervical spine flexion and increased the likelihood of fracture or dislocation between C4 and C7 [20]. Since the earlier work highlighted the importance of mechanical end-conditions on injury type, Myers et al. performed a controlled parametric study to examine the effects of end-conditions and determined that the neck could not be broken in flexion bending, but could be consistently dislocated in compression with constrained head rotation – an end condition similar to the one applied by Bauze and Ardran. To date, the 1991 Myers study is the only one to consistently produce the elusive BFD and it definitively precluded forced flexion of the head as a mechanism. In this same time frame, Dr. Torg was hypothesizing that compressive buckling in a straightened neck was the BFD mechanism. This was based on his review of injuries and film of football players [17, 82]. In fact, Dr. Torg and his colleagues produced the buckling kinematics and failure in a skeleton model [13]. In the mid 1990s, Nightingale et al. applied varied head constraints in compressive impact tests on cadavers and were able to produce some bilateral facet dislocations. The mechanism for the bilateral facet dislocation is now thought to be combined loading at the segment level secondary to neck compression and buckling (Fig. 11.12). There are one or two anecdotal cases (e.g. a full-nelson hold, performed until muscles fatigued) where static flexion-tension loads have been reported to cause injury but these are a rare exception [83]. The role of “hyperflexion” as a mechanism for BFD is still being debated in the interesting and unusual circumstance of the rugby scrum [83, 84].
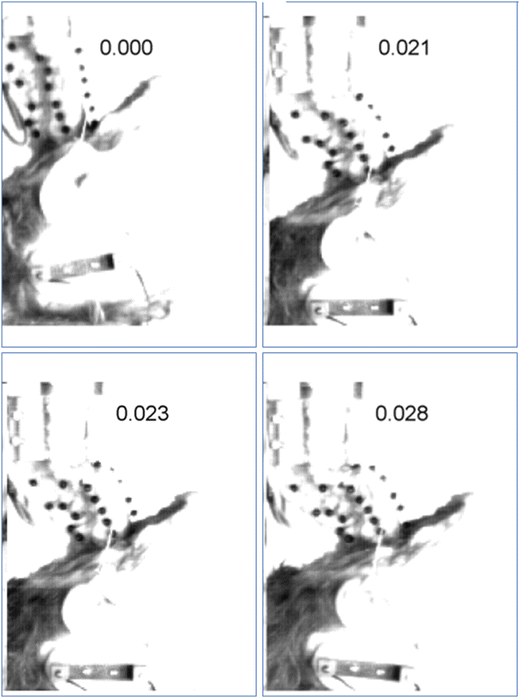
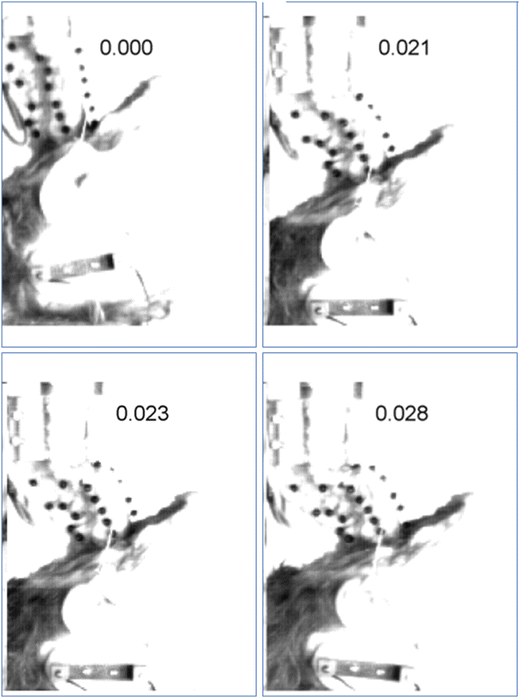
Fig. 11.12
Injury sequence showing first mode buckling and the initiation of a C6–C7 bilateral facet dislocation at 21 ms with full displacement at 28 ms. Note how little head rotation or translation occurs during this time period. The small amount of head rotation that does occur is in extension
11.4.2 Tension
Compared to compression, pure tensile injuries of the cervical spine are relatively rare. The development of active automotive restraint systems and increased seat belt use over the last three decades has markedly reduced the overall fatality and injury rates in automotive accidents. However, these technologies have given rise to a number of reported severe and fatal cervical spine injuries due to tensile mechanisms. These occur primarily during noncontact head decelerations and out-of-position airbag deployments. There is also some concern for inertial injuries to rear-seated occupants [85]. Inertial injuries typically have a significant component of flexion or extension and will be discussed further in Sect. 11.4.4. The research reviewed in this section is most relevant to out-of-position airbag deployments, but is also important in inertial loading.
Tensile experiments on the cervical spine have been performed by relatively few investigators. In 1971, Mertz and Patrick proposed a tensile tolerance of 1,135 N based on a single volunteer test [86]. This noninjurious static strength was offered as a lower bound for the corresponding dynamic strength. Sances et al. reported results obtained from tests on human ligamentous spines under tensile displacement [87]. Three specimens were tested and failed at a loading rate of 0.13 cm/min with failure forces ranging from 1,446 to 1,940 N and total distractions of 1.14–3.0 cm.
Yoganandan et al. reported on the experiments of Sances et al. and added the results of additional tests [88]. The first set of tests was performed on 20 isolated discs from eight cadavers (C2–C3, C3–C4, C4–C5, C5–C6, C7–T1). The average failure load was 569 ± 54 N at a displacement of 11.1 ± 1.1 mm. A second set of experiments reported by the authors was conducted on four isolated ligamentous spine preparations from skull to T3. Failure loads ranged from 800 to 1,900 N with a mean of 1,555 ± 459 N at a mean displacement of 27.1 ± 4.7 mm. All four specimens disarticulated at the C6–C7 level. The authors also performed a series of tests on three whole cadavers using a shoulder yoke and cable setup to apply tensile loads to the head. Only upper cervical spinal injuries were observed, including odontoid fractures and a basilar skull fracture. The mean failure load was 3,373 ± 464 N at an average distraction of 21.3 ± 1.9 mm. However, the head end condition was not well described in these tests.
In 2000, Van Ee at al published a systematic study of cervical spine stiffness and tolerance in tension [89]. Structural tests were conducted on six whole cervical spines of mixed sex. The specimens were separated into motion segments for component and failure testing. The strength of the upper cervical spine was 2,400 ± 270 N and was significantly stronger that the lower cervical spine (1,780 ± 230 N). The injuries in the lower cervical spine were all complete joint disruptions without bony fractures. The upper cervical spine injuries were O–C1 or C1–C2 dislocations and one Type II odontoid fracture. The strength and stiffness results were used in a computational model that accounted for the contributions of the cervical musculature. The muscle increased the tensile tolerance to 4,200 for full activation of all neck muscles.
Chancey et al., used optimization to estimate physiologically appropriate muscle activation levels for relaxed (sufficient to hold the head upright in equilibrium) and tensed (maximal activation without head motion) neck muscles [90]. Head and neck tension simulations under these activations showed that the muscles significantly affect tensile tolerance. Even though the ligamentous cervical spine will fail at a tensile load of 1.8 kN, the muscles increase the overall neck tolerance to between 3.1 and 3.7 kN for the relaxed and tensed states, respectively.
More recently, Dibb et al., performed a series of experiments on whole and segmented adult male cadavers [91]. Twenty male cervical spines were loaded in tension with a variety of end conditions and loading points (the anterior-posterior position of a vertical load relative to the occipital condyles). Cervical spine stiffness was found to be sensitive to both end condition and the loading point. Loading points that are eccentric from the condyles allow more head rotation and a more compliant response. As in prior studies, the upper cervical spine was found to be stronger in tension (2.1 ± 0.3 kN) than the lower cervical spine (1.6 ± 0.3 kN and 1.4 ± 0.3 kN for C4–C5 and C6–C7 respectively). The tensile strength of the upper cervical spine increased with a more anterior eccentric axial load, which is contrary to elementary beam theory. The authors hypothesized that this is due to the unique anatomy of the upper cervical spine which includes robust ligaments located anterior to the condyles. With loading aligned with those ligaments, they act in parallel and strength is increased over more posterior loading. In addition to O–C1 and C1–C2 dislocations, failures of the upper cervical spine included type III odontoid fractures (n = 6), basilar skull fractures (n = 2), occipital condyle fractures, and vertebral body fractures. Failure of the C4–C5 and C6–C7 also produced complete joints disruptions with some vertebral body and spinous process fractures originating near the fixation. No Hangman’s fractures were produced, but this may be due to the fixation of C2 and C3.
11.4.2.1 Discussion
Interest in the tensile tolerance of the neck was primarily sparked by a string of severe and fatal injuries to children and adults in low speed frontal crashes with airbag deployments. In 1991, a NHTSA Special Crash Investigation was initiated in response and it was determined that most of the victims were either not wearing belts or were very close to the airbag when it deployed. The mechanism of injury was thought to be tension and extension in the neck caused by interaction with a deploying airbag under the chin. The resulting injuries were basilar skull fractures, occipito-atlantal dislocations, and C1–C2 injuries [92–97].
One of the most interesting findings of the ensuing research on tension was that the upper cervical spine is stronger than the lower cervical spine. However this is not consistent with the field studies above in which upper cervical spine injuries are much more common. As a segmented column, one would expect that the weakest point in the neck would be the site of failure, but the findings of the NHTSA investigation showed a large number of upper cervical spine injuries in both adults and children. Chancey et al., found that this discrepancy is most likely due to the effects of the active musculature [90]. The muscles of the neck share tensile loads with the cervical spine by providing a parallel load path. Such load sharing increases the overall strength and stability of the neck and provides greater protection to the caudal motion segments because of the larger size and number of muscles in the lower cervical spine. So, here is a situation where the cadaver, without the benefit of a biofidelic computational model of the muscles, is not a good surrogate for the living human and this has implications for both tension experiments and the deceleration experiments discussed in Sect. 11.4.4.1.
Finally, the epidemiology and the experimental research show that tensile neck injuries in the lower cervical spine are rare. Since tension produces primarily upper cervical spine injuries, further discussion of these will be left to Sect. 11.4.5.
11.4.3 Torsion
Torsion has been thought to be a principal cause of both upper and lower cervical injury and dislocation; specifically unilateral facet dislocations and rotatary atlantoaxial dislocations. Types of atlantoaxial subluxations include unilateral, as well as bilateral, anterior and posterior subluxations [98]. These injuries refer to the dislocation of one or both of the atlas facet surfaces on the axis facet joint surface and are thought to represent combinations of shear and torsion [38].
Cadaver studies have shown that torsional loading of the head can produce rotary atlantoaxial dislocation, with or without tearing of the alar ligaments [99, 100]. Estimates of the lower bound of torsional tolerance are available from Myers et al. [101]. Axial torque of 17.2 ± 5.1 Nm produced upper cervical injury in whole cervical spines (occiput to T1). Goel et al. report a slightly lower value of 13.6 ± 4.5 Nm in isolated upper cervical spine segments; however, the rates of loading in these experiments were considerably lower than in the Myers study (4°/s vs. 500°/s). Extrapolating piece-wise linear torsional stiffness data from volunteer decelerations reported by Wismans and Spenny to the 114 ± 6.3° of rotation required to produce injury in the cadaver, we obtain an estimate of human torsional tolerance of approximately 28 N-m [102].
11.4.3.1 Discussion
Myers et al. showed that the lower cervical spine is stronger in torsion than the atlantoaxial joint, mitigating torsion as a primary cause of lower cervical injury [67]. The notion that torsion can predispose the lower cervical spine to injury from other types of loading (i.e., flexion), while a common belief, is clearly not true for catastrophic injury (though it does play a role in whiplash) [103].
The etiology of unilateral facet dislocations has been the subject of interest and controversy. Huelke et al. and Harris et al. reported that unilateral dislocation was the result of combined flexion and rotation [2, 42]. Gosch et al. reported that in purely anterior to posterior deceleration sled tests of primates, axial rotation was observed and unilateral facet dislocation produced [104]. Roaf reported that torsion was required to produce lower cervical ligamentous injury and dislocation and that hyperflexion alone could not produce ligamentous injury [80]. Rogers and White and Panjabi noted that unilateral dislocation resulted from an exaggeration of the normal coupling of lower cervical lateral bending and rotation but did not describe the loads required to produce the injury [38, 105]. Braakman and Vinken suggested that unilateral facet dislocation was the result of combined flexion and rotation [33]. Torg and Otis stated that the lesion was the result of compression and buckling [13, 14, 17]. Bauze and Ardran produced unilateral facet dislocations in a number of specimens loaded in compression and flexion [66]. Their apparatus applied no torque to the specimen, and yet lower cervical rotation was observed prior to unilateral dislocation. Myers et al. demonstrated that while unilateral facet dislocation could be produced by direct torsional loading of the lower cervical spine, the lesion could not be the result of torsional loading of the head because of the comparative weakness of the atlantoaxial joint [100]. The authors currently believe that the lesion is produced by a mechanism similar to the bilateral facet dislocation, with the difference being due to bending out of the plane of symmetry, or the presence of pre-existing facet tropism of other structural asymmetry. Another possibility is that the unilateral facet dislocation is a partially reduced bilateral facet dislocation, which might account for the cohort of these lesions that have complete spinal cord injury [33].
11.4.4 Sagittal Plane Bending
Cervical spine bending has long been thought to play a major role in injury mechanisms. However, recent research calls this into question, particularly in flexion. The research on neck bending falls into two broad categories. The first is deceleration tests designed to create inertial loads similar to those in fore and aft impacts. These produce either flexion or extension with a large component of tension; therefore, they are not pure bending loads. The second category is isolated cervical spine tests in which the bending moment is pure. Until the turn of the millennium, while many orthopaedic studies has examined the kinematics of the cervical spine when subjected to pure moments, there had been very little research on tolerance to moment in the second category.
11.4.4.1 Inertial Loads
One of the most frequently cited studies is the work of Mertz and Patrick [86, 106]. They summarized data from forward and rearward sled decelerations of one human volunteer (Patrick) and four cadavers with weights added to the head at, above, or below the center of gravity of the head. Despite having extremely limited data, these authors were able to define corridors for the kinematic response and recommended noninjurious tolerance values in dynamic and static loading situations that proved useful in motor vehicle safety standards for many years. Reporting data from both volunteer and cadaver decelerations, the authors determined a lower bound for the risk for neck injury based on the bending moment estimated at the occipital condyles for flexion and extension loading. For extension, the authors report noninjurious loading of 35 ft-lb (47.3 Nm) with ligamentous injury expected above 42 ft-lb (56.7 Nm). For flexion, the authors report initiation of pain at 44.0 ft-lb (59.4 Nm), a maximum voluntary loading of 65 ft-lb (87.8 Nm), and the risk for structural injury above 140 ft-lb (189 Nm) in the cadaver following contact of the chin on the chest. The largest deceleration applied to a cadaver produced calculated moment of 189 Nm with no evidence of injury detected by x-rays. This limit was suggested as the upper bound of ligamentous spine tolerance in flexion, despite the lack of injury. The authors note however, that muscular injury may occur at loading below the latter value.
Bass et al. tested 36 head and neck preparations in frontal decelerations impacts to develop a predictive Neck Injury Index [107]. The tests produced AIS 3+ injuries in 11 of the specimens, with four injuries that were judged to have the potential for cord damage. Most of the injuries were to the soft tissues, with the osseous injuries being relatively minor. Upright orientation of the head and neck produced significantly less tension and shear (1,251 and 1,376 N, respectively) than orientations run with 30° of forward flexion (2,146 and 3,747 N, respectively) despite being run at significantly lower velocities. The authors suggest that this was because the neck was loaded in a stiffer mode when flexed forward (more tensile than bending). There was no significant difference in peak flexion moments.
Pintar et al. [108] subjected five cadavers to belted frontal impacts ranging in severity from 13 to 57 km/h and resulting in sled peak accelerations from 10 to 20 Gs. The instrumentation included accelerometer packs on the head and T1 as well as belt load sensors. Chin-to chest contact occurred in all tests, but after the peak calculated forces. The injuries produced in four of the five specimens were dislocations of the C7-T1 (n = 2) and dislocations of C6–C7 and T1–T2. The four specimens with cervical spine fractures also had multiple rib fractures and assorted fractures of the manubrium, clavicle and sternum. In the high velocity tests, the mean axial force and moment at the base of the neck were 1,424 N and 192 N-m respectively. At the occipital condyles they were 1,570 N and 41 N-m. The resultant force in both locations was not reported, but was substantially higher than the z component.
11.4.4.2 Bending Loads
The clinical finding of a spinal cord injury without radiographic abnormality (SCIWORA) by Taylor and Blackwood prompted interest in extension as a causative agent [109]. In a cadaver study by Taylor, which combined myelography with manual loading of the head in flexion and extension, demonstrated posterior canal defects in “forced” hyperextension. These defects were thought to be the result of bulging of the flaval ligaments into the neural canal and were produced without overt structural damage to the spine. The author postulated, based on this observation, that extension could produce spinal cord injury without trauma to the cervical spine. Further evaluation and the recognition of disc bulging during loading reinforced this as a possible mechanism of SCIWORA [110, 111]. The mechanism has also been observed in the more recent compressive impact testing of Saari et al. [72].
In a study by Gadd et al., four whole cadavers were loaded manually using levers coupled to the head to apply combined shear and bending (flexion-extension or lateral) [112]. Rotation in extension greater than 80° or 60° in lateral bending produced audible sounds in two of the four specimens and resulted in decreased stiffness following reapplication of the same load. Defining these events as minor injury, mean mid-cervical moment at injury was 24.3 ± 12 Nm.
The end condition study by Myers et al. in 1991 applied compressive displacements to whole cervical spines with a free rostral end condition [113]. This was not pure moment loading; however, the tests produced primarily flexion because of the limited ability of the cervical spine to react compression with an unconstrained head. The mean peak axial load was 289 ± 81 N with a mean axial displacement of 8.6 ± 1.2 cm and a mean head rotation of 96 ± 7°. None of the specimens were injured despite the large, anatomically impossible head rotations.
Nightingale et al. examined the flexibility and strength of 52 female motion segments in both flexion and extension [114]. This was followed in 2007 by a similar study of 41 male cervical spines [115]. Their flexibility results were similar to prior studies [116–118] and they were the first to provide statistically meaningful tolerance data in pure bending (Table 11.1). They found that the upper cervical spine was stronger than the lower cervical spine. For both males and females, the lower cervical spine failures were complete joint disruptions (tearing of all the ligaments and the disc) with a few associated avulsion fractures of the vertebral bodies. The most common injuries to the upper cervical spine were odontoid fractures, which occurred primarily in extension, but were also observed in flexion.
Wheeldon et al., 2006 tested whole lower cervical spines in pure flexion and extension [119]. The segments were from C2 to T1 and the range of motion for a 2.5 N-m moment was near 50° for flexion and near 30° for extension. The study was unusual in that the mean age of the donors was only 33 years old. By comparing their results with prior studies, the authors concluded that younger specimens were significantly less stiff in bending than older specimens. This was also the only study to use whole lower cervical spines and the large rotations encountered even without the O-C1 joint illustrate the technical difficulty in applying a pure follower moment to these very flexible structures.
11.4.4.3 Discussion
Bending studies on isolated cervical spine have shown that the neck is remarkably flexible in bending and its toughness in this mode of loading is underestimated. Researchers who have tried to fail the whole spine in this mode have not succeeded [113] and they have had to resort to segment tests. Even applying pure moments to isolated upper cervical spine segments is technically difficult and requires very specialized test equipment. Summing the angles at failure for the upper and lower cervical spine gives an estimate of the degree of head flexion required for a pure bending injury of the cervical spine. This value is greater than 120° and is clearly nonphysiologic as shown in Fig. 11.13. It can be stated with confidence that failure of the cervical spine in pure flexion is an anatomical impossibility, validating the hypothesis suggested decades ago by Roaf [66]. This may also be true of extension, although further modeling and experimental work is necessary to state this with similar confidence.
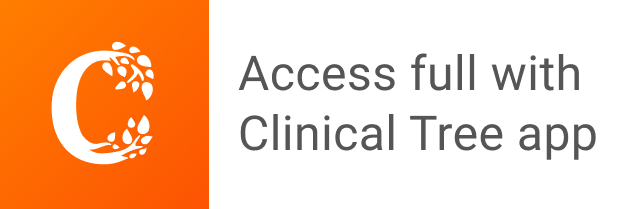