What is molecular imaging? The term certainly has been used to describe a wide variety of imaging approaches. For our purpose, we limit it to a definition so elegantly put forth by Massoud and Gambhir as “the visual representation, characterization, and quantification of biological processes at the cellular and subcellular levels within intact living organisms. It is a novel multidisciplinary field, in which the images produced reflect cellular and molecular pathways and in vivo mechanisms of disease present within the context of physiologically authentic environments.” With molecular imaging techniques we can study the spatiotemporal distribution of specific molecular entities, such as antibodies and cytokines, or we can track specific cell populations. We can study the expression of specific genes or the augmentation of certain gene products, such as proteases.
So, why do we need molecular imaging? Using tried-and-true methods of radiography, MRI, computed tomography, or a combination of these, we can usually establish the diagnosis, or so it seems. The current methods, however, have weaknesses with regard to the assessment of chronic diseases, detection of acute-on-chronic changes (whether related to disease recurrence or post-therapeutic effects), and identification of hyperacute phases of disease. It is in this arena of uncertainty that molecular imaging may prove very useful, providing information not discernible by current clinical imaging techniques.
Furthermore, the fusion of functional and molecular imaging procedures with conventional, anatomic imaging methods will no doubt add to the specificity and sensitivity of imaging diagnosis, just as 2-deoxy-2-( 18 F)fluoro -d -deoxyglucose (FDG)-positron emission tomography (PET)/CT has done for general tumor screening and staging. Molecular imaging procedures should be viewed as important complementary tools to an already powerful armamentarium of clinical imaging devices. For the near future, hybrid imaging technologies such as PET/CT, single photon emissions computed tomography/CT (SPECT/CT), and, eventually, PET/MRI will be the workhorses for the molecular imager. New molecular “contrast agents” for MRI and ultrasonography will further enhance their potential. Additionally, commonly used optical imaging methods used in preclinical animal models of disease, namely, fluorescence and bioluminescence molecular imaging, will also make strides into the clinical realm.
Perhaps another important reason to increase one’s understanding of this evolving discipline of molecular imaging is that in this era of molecular medicine, therapies, including gene therapy and cell-based therapies, can be directly and indirectly studied using molecular imaging procedures and techniques. Basic scientists and molecular imagers are combining forces to find better ways to design and monitor such therapy in living subjects. Ultimately, clinical imagers will be asked to determine whether a certain gene or cell therapy is working in the targeted location in the manner in which it was designed.
Although the application of molecular imaging procedures runs the gamut of musculoskeletal diseases, our discussion of molecular imaging procedures and techniques in this chapter relates its application to two important chronic musculoskeletal disease entities: rheumatoid arthritis (RA) and osteoarthritis (OA).
RHEUMATOID ARTHRITIS
Current Imaging Challenges
Despite the improved sensitivity of newer methods, conventional radiography remains the dominant imaging method for RA evaluation. Radiography can provide excellent detail of osseous erosions, joint space narrowing, and periarticular osteopenia, but only after the clinical symptoms have been present for several months or even years. Furthermore, radiography is considered a relatively insensitive means to measure changes of RA. Conventional radiography may appear negative for a period of 6 to 12 months after the onset of disease, and in as many as 25% of RA patients no radiographic erosions are seen for as long as 5 years. The lack of radiologic erosions has unfortunately been used to exclude patients from clinical trials or the initiation of antirheumatic drugs.
Patients suffering from RA would greatly benefit from early detection and treatment of disease. MRI and ultrasonography are being used successfully to detect early RA-related changes in patients and preclinical animal models. MRI allows early detection of osseous and soft tissue changes in RA, including joint effusions, synovitis, tenosynovitis, tendon/ligament damage, bone marrow signal changes, and cartilage destruction; and it is currently considered the superior method in the evaluation of this disease. MRI can be applied to the analysis of relatively early changes and chronic periods of the disease. Gadolinium-enhanced MRI allows detection of synovitis with great sensitivity and aids in the differentiation of soft tissue inflammation and a joint effusion.
High-frequency ultrasonography (7.5-10 MHz for conventional studies and 20 MHz for fine detail musculoskeletal work), which can detect synovial thickening, and power Doppler ultrasonography, which indirectly measures hyperemia related to capillary flow, have been used to assess the thickened, hypervascularized synovium of the inflamed small joints of the hands and feet in RA. Ultrasound evaluation is believed to have superior sensitivity to MRI with regard to the detection of effusions and tenosynovial fluid but is inferior to MRI in the assessment of synovitis, tendon/ligament damage, and bone erosions. Ultrasonography is particularly useful during earlier stages of disease and serves as a relatively accessible and inexpensive procedure using nonionizing radiation to assess small joints.
Radionuclide techniques can also be used to detect early changes of RA. By specifically “tagging” or labeling important cellular and protein mediators, RA can be “visualized” from a cellular or molecular context (see later). The white blood cell (WBC) or leukocyte scan, for example, is a classic assay for studying the migration and trafficking of these specialized cells to sites of inflammation. Studies of rheumatoid arthritic wrists have shown that MR measurements of synovial volume correlated with the metabolic rate of the inflamed tissue as measured by FDG-PET ( r > .86; P < .0001). Further details on the utility of FDG-PET have been provided in Chapter 7 .
Ultimately, the conventional imaging methods just described can only demonstrate abnormalities that are relatively downstream to the inciting event, that is, activation of the T cell. Effusions, hypervascularity, erosions, and synovial proliferation are changes seen only after the activation of T cells and macrophages. Molecular imaging protocols, as discussed later in this chapter, attempt to image these cells and related acute mediators of disease ( Fig. 9-1A ).


Pathogenesis
Despite extensive reviews on the subject, the cause of RA remains a mystery. Presumably, an unidentified arthritogenic antigen stimulates or activates CD4 + T cells that normally reside in synovium. Neighboring cells, such as dendritic cells, monocytes, synovial fibroblasts, and macrophages, are induced to produce the proinflammatory cytokines, interleukin (IL)-1 (IL-1), tumor necrosis factor-α (TNF-α) and IL-6. These same cells are also stimulated to secrete the matrix metalloproteinases, which cause degradation of the synovium and cartilaginous and osseous extracellular matrix tissue. Activated T cells also stimulate B cells to produce immunoglobulins, the most notorious of which is rheumatoid factor (RF). RF may play a role in the pathogenesis of RA by binding and activating complement, which further contributes to the inflammatory process. Furthermore, activated T cells also possess the ability to directly activate osteoclasts, which are cells that are responsible for the bone erosions typical of the disease. Angiogenesis, an eventual prominent feature in the synovial tissues, can be attributed to activated macrophages, lymphocytes, and fibroblasts. It is this proliferation of vascular elements that gives rise to the synovial enhancement seen with gadolinium-enhanced MRI and power Doppler ultrasonography. Endothelial cells that populate both the newly created and preexisting vessels produce proinflammatory proteins (e.g., certain receptors) and adhesion molecules under inflammatory conditions. It is these increased cytokines, proteinases, immunoglobulins, inflammatory mediators, adhesion molecules, and other molecules that molecular imaging protocols exploit for generating image contrast.
Molecular Imaging
A fundamental explanation for the frustration of clinicians in defining appropriate therapeutic options in patients with RA is the variable clinical manifestations and responses to treatment. Adding to the complexity of this disease are the seemingly uncoupled events of synovitis and bone destruction. For these reasons, clinicians and molecular imagers alike are interested in finding consistent, reliable biomarkers of RA. Identifying common cellular and molecular denominators of disease that can be applied broadly during the course of disease is of great importance. Rather than focusing on anatomic changes of the disease, molecular imaging attempts to detect cellular and molecular perturbations by exploiting the stereotypic changes seen in this disease. Inflammatory cells, for example, are recruited to an affected joint, an event that, in turn, is followed by a cascade of inflammation-related biochemical processes such as the upregulation of certain cell surface receptors. By specifically “tagging” inflammatory cells or carefully selected ligands, one may be able to define specific and discrete aspects of the inflammatory process. Furthermore, by tailoring molecular imaging protocols in light of current molecular therapies, we may be able to better assist our colleagues who are designing therapies specifically to deal with the action of T cells, autoantibodies, cytokines, or other effector cells. A summary of both conventional and molecular methods to image RA is provided in Table 9-1 .
Targeted Pathology | Temporal Pattern | Modality and Specific Methods | Clinical vs. Preclinical | References |
---|---|---|---|---|
T-cell infiltration | Hyperacute to acute | NM: 99m Tc-HMPAO-labeled T cells | Clinical | |
PET reporter genes | Preclinical | |||
Optical: luciferase reporter genes | Preclinical | |||
MRI: CLIO-Tat | Preclinical | |||
Antibody-based Imaging | Hyperacute to chronic | NM: 64 Cu-labeled anti-GPI | Preclinical | |
111 In- or 99m Tc-labeled nonspecific IgG | Clinical | |||
99m Tc-labeled anti-CD3 | Clinical | |||
99m Tc-labeled anti-CD4 | Clinical | |||
111 In- or 99mTc-labeled anti-E-selectin Fab | Clinical | |||
Radiolabeled anti-TNF-α, anti-IL-1, anti-IL-2, and anti-IL-6 | Preclinical | |||
123 I-antileukoproteinase | Preclinical | |||
99m Tc-labeled anti-CD15 immunoglobulin M (IgM) | Clinical | |||
Optical: NIRF-labeled anti-F4/80 macrophage surface antigen | Preclinical | |||
Macrophage recruitment | Hyperacute to chronic | MRI: Superparamagnetic iron oxide particles (SPIO) | Clinical | |
Ultrasmall SPIO (USPIO) | Preclinical | |||
NM: 99m Tc-folic acid (EC20; folate receptor imaging agent) | Preclinical | |||
Optical: NIRF-labeled folate (NIR2-folate) | Preclinical | |||
Leukocyte recruitment | Hyperacute to chronic | NM: 99m Tc-HMPAO-labeled WBCs | Clinical | |
111 In-labeled WBC | Clinical | |||
67 Ga-citrate | Clinical | |||
Cytokine production | Hyperacute to chronic | NM: 123 I-labeled interleukin-1 receptor antagonist (IL-1ra) | Clinical | |
Activated nuclear factor-κB (NF-κB) | Hyperacute to CHRONIC | Optical: NF-κB reporter mouse | Preclinical | , |
Matrix-degrading enzymes | Hyperacute to chronic | Optical: cathepsin and matrix metalloproteinase-sensitive NIRFs | Preclinical | , |
Synovitis/ tenosynovitis | Acute to chronic | US: Gray-scale sonography Power Doppler | Clinical | |
MRI: Nonenhanced and gadolinium-enhanced MRI | Clinical | |||
Joint effusion | Acute to chronic | Ultrasonography | Clinical | |
MRI | Clinical | |||
Radiography | Clinical | |||
Soft tissue swelling | Acute to chronic | Ultrasonography | Clinical | |
MRI | Clinical | |||
Radiography | Clinical | |||
Bone marrow changes | Acute to chronic | MRI | Clinical | |
NM: 99m Tc-methylene diphosphonate (MDP) | Clinical | |||
Increased metabolism | Acute to chronic | NM: 18 F-FDG-PET | Clinical | |
Cell stress/apoptosis | Acute to chronic | NM: 99m Tc-, 123 I-, 124 I, 125 I-, 18 F-, 11 C-, 64 Cu-, 67 Ga-, and 68 Ga-labeled annexin V | Clinical | |
MRI: CLIO-labeled annexin V | Clinical | |||
Optical: Cy5.5-labeled annexin V | Clinical | |||
Vascular inflammation angiogenesis | Acute to chronic | NM: 99m Tc-anti-E-selectin-Fab | Preclinical | |
Power Doppler ultrasonography | Clinical | |||
MRI: gadolinium-enhanced | Clinical | |||
Osseous or cartilaginous erosions | Intermediate to chronic | Radiography | Clinical | |
CT | Clinical | |||
MRI | Clinical | |||
Tendon damage | Intermediate to chronic | Ultrasonography | Clinical | |
MRI | Clinical | |||
Subluxations and dislocations | Chronic | Radiography | Clinical | |
CT | Clinical | |||
MRI | Clinical | |||
Secondary OA | Chronic | Radiography | Clinical | |
CT | Clinical | |||
MRI | Clinical |
TRACKING INFLAMMATORY CELLS AND SPECIFIC SUBPOPULATIONS
Cell trafficking of specific cell populations to inflamed joints is a common strategy to image RA and related autoimmune diseases. This concept is the underlying premise for many long-established radionuclide-based techniques such as the gallium-67 ( 67 Ga) scan and the WBC scan. How PET-, optical-, and MRI-based methods compare with standard methods (e.g., the WBC scan) remains to be seen because head-to-head comparisons have not been performed. Generalized methods for improving radiolabeling of T lymphocytes and monocytes for PET imaging have been described.
T-CELL TRAFFICKING
One particularly exciting method to monitor the pathogenesis of RA will be to track one of the main cellular culprits associated with this autoimmune disease, namely, the reactive CD4 + T-cell lymphocytes. There are means of isolating and purifying such cells from a subject using sophisticated cell-sorting techniques. Once isolated from peripheral blood, these cells can be “marked” with a contrast agent and then reintroduced into the same subject. After a period of incubation, the subject can undergo serial imaging to determine the distribution of the cells. Traditional cell-labeling techniques are based on radionuclide methods (e.g., 111 In-oxime, 111 In-tropolonate, and 99m Tc-HMPAO) ; however, a number of PET, optical, and MR-compatible methods are currently available.
In animal models of collagen-induced RA, the localization of collagen-reactive CD4 + cells has been shown through imaging assays in living mice. To track the cells, the investigators transfected reactive CD4 + T-cell hybridomas with a recombinant retroviral vector encoding green fluorescent protein (GFP) and firefly luciferase optical-based reporter genes. A detailed review of in-vivo reporter genes is beyond the scope of this chapter but can be found elsewhere. Briefly, cells such as the T cells have been genetically re-engineered to express fluorescent or bioluminescence proteins, which give these “labeled” cells the ability to emit light under the appropriate conditions. Light emission from the T cells can be detected with a highly sensitive cooled, optical, charged couple device (CCD) camera, resulting in images that give the general spatial location of these optically labeled T cells. Images obtained from nonarthritic, control animals showed that optically labeled T cells resided in axillary and inguinal lymph nodes (see Fig. 9-1B ). By comparison, animals with polyarticular inflammation showed that the specially marked cells migrated from the lymph nodes to the inflamed joints. Investigators also used these cells to specifically deliver anti-inflammatory gene therapy to the joints in the form of cytokine antagonist IL-12 p40. Injection of collagen-specific IL-12 p40–producing T cells retarded the development of collagen-induced arthritis.
Another method to monitor T-cell traffic has been developed for MRI. T cells isolated from a subject can be loaded with dextran-coated superparamagnetic iron oxide particles (SPIO) or similar dextran-coated crosslinked iron oxide particles (CLIO). When exposed to SPIO, T-cells will engulf the 30-nm particles by endocytosis. The T cells are eventually reintroduced into the subject, and the subject is scanned. On gradient-echo sequences, cells carrying this contrast agent appear low in signal intensity owing to the large susceptibility effect generated by the sequestered SPIO particles. In rat models of cardiac, renal, and lung allograft rejection, migration of SPIO-labeled T cells to the allograft has been found during rejection.
Recently, this cell-labeling contrast agent has undergone a modification that facilitates its entry into target cells. The superparamagnetic core of the iron oxide particle has been linked with peptide sequences of the transactivator protein (Tat) of human immunodeficiency virus-1 (HIV-1), resulting in CLIO-Tat particles. The HIV-1 Tat protein is an 86-amino acid protein required for replication of the virus. It appears to be involved in translocating the viral particle freely across cellular and nuclear membranes. A short segment of the peptide, amino acid residues 48-57, is largely responsible for this transport phenomenon, and when this short peptide segment is attached to a CLIO particle it increases the cell labeling efficiency 100-fold. This agent has been tested in animals, in which the expected migration of CLIO-Tat–labeled T cells to the reticuloendothelial system is seen, and in an animal model of autoimmune diabetes, in which labeled T cells traffic to the pancreas. Other recent cell-labeling techniques involve directly labeling cells with technetium-99m ( 99m Tc) or with MRI-detectable DNA-binding chelates. Many of these methods have not yet been applied to arthritic models but have great potential.
MACROPHAGE TRAFFICKING
SPIO can also be used to monitor monocytic/macrophage migration patterns in the setting of RA. After intravenous injection of SPIO particles, cells that reside in the reticuloendothelial syndrome, including macrophages, engulf the agent. Because macrophages are recruited to inflamed joints, monitoring their distribution by SPIO-based techniques can be helpful, especially during early phases of the disease. MRI can be used to study the migration of these cells from the reticuloendothelial syndrome to inflamed joints. Investigators have successfully documented the migration of SPIO-labeled macrophages to the synovium of a rat model of RA (see Fig. 9-1C ).
Activated macrophages are also known to express a large number of folic acid receptors, and this characteristic has been exploited by several investigators as a means to study the distribution of macrophages in the inflamed joints of animals. The ligand for the receptor, folic acid, has been coupled to a variety of optical and radionuclide labels, and early studies with the intravenously administered, labeled folate in animal models have shown localization of the tracer in inflamed joints.
Leukocyte Trafficking
Leukocytes include neutrophils, basophils, eosinophils, monocytes, macrophages, and lymphocytes. They participate in a host of inflammatory reactions in disease such as RA, infection, and trauma. Imaging leukocyte trafficking has been a staple procedure among the radionuclide-based imaging examinations for the past few decades. Better known as a WBC scan, a variety of methods have been developed that can successfully label leukocytes with 99m Tc, 67 Ga, and indium-111 ( 111 In), and these methods have been specifically applied to the study of RA. Because of its relatively low cost, ease of use, low radiation burden, general accessibility, and high sensitivity and the significant correlation of accumulated activity at sites of active disease (as measured by the swollen joint count), 99m Tc-hexamethylpropyleneamine oxime (HMPAO)–labeled leukocyte scintigraphy is one of the methods of choice to study migration of these cells into inflamed joints.
ANTIBODY-BASED AND CYTOKINE-BASED IMAGING
Autoantibodies that develop during the course of RA have been labeled and injected, and their distribution has been studied. Additionally, specific antibodies to T cells, general leukocytes, cytokines, and receptors have also been utilized in this manner. A variety of specific and nonspecific antibodies of interest have been radiolabeled or fluorescently labeled and imaged in animal models of RA (see Table 9-1 ). One example is an antibody to the glycolysis enzyme glucose-6-phosphate isomerase (GPI), which has been the subject of intense interest, because 50% of RA patients have serum antibodies to GPI. By labeling anti-GPI immunoglobulin G (IgG) with the positron emitter copper-64 ( 64 Cu), investigators have studied the biodistribution of this antibody in animal models of RA. PET imaging reveals accumulation of the labeled antibody in the front paws, hind ankles, and rear feet of animals with RA, while control animals showed negligible articular uptake. Other antibodies, including polyclonal, nonspecific IgG, have been similarly interrogated using scintigraphic methods. Whereas the exact mechanism remains poorly understood, the use of nonspecific antibodies is encouraged by its high sensitivity. However, the lack of specificity (<50%) and the relative high cost compared with more conventional scintigraphic techniques (e.g., leukocyte scintigraphy) probably prevent the routine utilization of radiolabeled antibody–based techniques at the current time.
Another way to track antibodies is through fluorescence labeling. Using a fluorescently labeled (Cy5.5) monoclonal antibody to F4/80 antigen, investigators have demonstrated the localization of this antibody in the inflamed joints of mice using optical imaging strategies (see Fig. 9-1D ). The antigen F4/80 is present on the surface of macrophages that infiltrate an inflamed joint, and targeted imaging of these macrophages via antibodies represents yet another approach to RA imaging. Numerous other antibodies (see Table 9-1 ) have been developed to bind to the various mediators of disease, including T cells, cytokines, and receptors. Recent evidence, however, suggests that the use of antibody-based imaging techniques may be problematic, because only a fraction of the radiolabeled monoclonal antibodies (directed CD4 + T lymphocytes) actually bind to target cells while a majority of the dose gets trapped in the reticuloendothelial system such as the liver. Modifications to antibodies (i.e., diabodies, tetrabodies, and minibodies) are currently being studied to avoid such problems in the future.
Just as antibodies can be labeled, so can inflammatory cytokines or receptor antagonists. Investigators have labeled a receptor antagonist to the interleukin-1 receptor (IL-1ra) with iodine-123, and the tracer has been tested in humans. Gamma camera images show localization of the tracer to affected joints in patients with RA (see Fig. 9-1E ).
ACTIVATED NUCLEAR FACTOR-κ B (NF-κB) IMAGING
NF-κB is a rapid-acting transcription factor found in nearly all cell types and is a major proinflammatory mediator. Activation of NF-κB stimulates or regulates transcription of over 150 genes, including monocyte chemotactic protein 1, intracellular adhesion molecule (ICAM-1), TNF-α, IL-1β, IL-6, inducible nitric oxide synthase (iNOS), IL-8, matrix metalloproteinases, and cyclooxygenase 2 (COX-2), which are necessary for the initialization and persistence of chronic inflammation, including that seen in RA. Prior to modulating the expression of these genes, activation of NF-κB requires enzymatic action with the IkB kinase (IKK) complex. After activation, NF-κB translocates into the nucleus and binds to a 10-base-pair DNA sequence. Under the control of the NF-κB–inducible promoter, transgenic mice have been developed that express luciferase under the control of an activated NF-κB, permitting real-time, in-vivo longitudinal, optical imaging of NF-κB activity in living animals. This “NF-κB reporter mouse” has already demonstrated its prowess as an important tool in monitoring multicellular inflammatory responses in models of arthritis and studies of drug action. Through its selective inhibitory effect on the IKK complex, ML120B, a potent, small molecule, for example, has been shown to reduce NF-κB activity and concomitantly reduce the expression of multiple inflammation genes in the NF-κB pathway in an antibody-induced arthritis model.
PROTEASE-SPECIFIC ACTIVATED NEAR-INFRARED PROBES
The production of matrix metalloproteinases (MMP) and cathepsins is also enhanced in active stages of joint inflammation. In the optical imaging world, a recently developed “smart” fluorescent probe has been developed to detect elevated levels of MMP and cathepsins. This probe, or contrast agent, will emit light only when cleaved by its specific target enzyme; otherwise, the probe is optically invisible. This breed of fluorescent probe is dependent on the close proximity of multiple Cy5.5 near-infrared fluorochromes (NIRF), which are bound to a synthetic graft copolymer backbone (partially methoxypoly[ethylene glycol] modified poly -l -lysine).
When placed in close proximity, a pair of these fluorochromes will “quench” each other and, therefore, be “invisible.” Once the fluorochromes achieve a minimal physical separation, they will begin to fluoresce. The basis of this physical phenomenon is called mutual fluorescence energy transfer (FRET). When cathepsin B, which has lysine-lysine substrate specificity, interacts with the Cy5.5-loaded NIRF “contrast agent,” the lysine backbone is cleaved and the Cy5.5 fluorochromes spatially dissociate to fluoresce. These contrast agents are “red shifted,” and, thus, fluoresce in the near-infrared portion of the electromagnetic spectrum (excitation wavelength of 673 nm; emissions wavelength of 689 nm). Red-shifted fluorochromes are ideal fluorescent agents for use in intact organisms, because longer wavelengths penetrate tissues such as bone better than green-fluorescing agents.
Because many of the arthritides (as well as tumors) during stages of progression and angiogenesis necessarily elaborate certain matrix-digesting proteases, these clever, or “smart,” biocompatible autoquenched near-infrared fluorescent probes can potentially be used to detect a variety of arthritic processes (including OA), as well as cancers. The successful application of a cathepsin B–sensitive probe has been demonstrated in an animal model of RA. After intravenous administration of the NIRF probe, light was seen to arise from the inflamed knees and ankles of animals with RA but not from the joints of normal animals.
CELL STRESS/APOPTOSIS IMAGING
The inflammation of RA is an event that is stressful to cells involved in the process. Furthermore, there appears to be an abnormal rate of apoptosis occurring in synovial tissue in RA. Whether the level of apoptosis is excessive or reduced remains a matter of debate. Imaging of cell stress and apoptosis is now possible in living subjects using an endogenous molecule called annexin V. Annexin V recognizes a cell membrane component that presents itself during times of increased metabolic stress, apoptosis, and necrosis (see later).
In the collagen-induced arthritis model, increased uptake of radiolabeled 99m Tc-HYNIC-annexin V is seen in the paws of arthritic animals when compared with controls. Steroid treatment of the arthritic model not only alleviates clinical manifestations of collagen-induced arthritis but also results in diminished uptake of annexin V, suggesting that the steroids provided some type of cytoprotective effect. In another example, near-infrared fluorescence (NIRF) imaging with Cy5.5-annexin V showed no significant imaging differences between collagen-induced arthritis models and controls (see Fig. 9-1F ). When methotrexate was administered to both groups, an increased accumulation of Cy5.5-annexin V was observed in the joints of both models, with substantially more seen in the collagen-induced arthritis model.
VASCULAR MARKERS
Vascular endothelial cells associated with the area of inflammation, whether related to trauma, infection, arthritis, or other causes, become “activated,” and their activation is integral to the process of leukocytic recruitment and ensuing synovitis. These stimulated endothelial cells necessarily amplify a number of cellular adhesion molecules, including selectins (e.g., P-selectin, E-selectin), integrins (e.g., β 4 , β 1 ), immunoglobulins (e.g., ICAM-1), vascular cellular adhesion molecule-1 (VCAM-1), and cadherins (e.g., E-cadherin, cadherin-11), to attract and bind inflammatory cells. Additionally, the endothelial cell–specific vascular endothelial growth factor (VEGF), which has been well characterized in hypoxic stress models and tumor angiogenesis, has also been found to be augmented in aggressive synovitis and strongly correlates with RA activity.
In efforts to improve detection of vasculitic processes, a number of contrast agents that target endothelial epitopes have been developed for ultrasonography, MRI, and radionuclide-based imaging. For example, echogenic microbubbles have been conjugated to antibodies for ICAM-1, fibrinogen, or P-selectin. These agents will localize to the endothelium of an organ that has sustained an ischemia-reperfusion injury. Alternatively, these agents will diffusely localize to the endothelium when systemic tumor TNF-α is administered. Echogenic liposomal agents have also been coated with anti–ICAM-1, resulting in their localization to atheromatous plaques during ultrasound imaging. Radionuclide approaches that employ a 99m Tc-radiolabeled E-selectin–binding peptide have also been attempted with some success. Optical approaches have employed highly fluorescent quantum dots that have antibodies directed against adhesion molecules conjugated to its surface. This contrast agent has successfully highlighted retinal vascular inflammation seen in a rat model of diabetes.
MR researchers have also created agents for cellular adhesion molecules. One group of investigators has produced liposomes containing a gadolinium lipid chelator that becomes paramagnetic in the presence of gadolinium. By biotinylating these paramagnetic liposomes, biotinylated antibodies can be conjugated to the liposomes via an avidin linker, creating an antibody-conjugated paramagnetic liposome (ACPL). In an animal model of experimental autoimmune encephalitis (EAE), localization of ICAM-1 ACPL in the brain leads to increased signal intensity. Another MR-based imaging agent involves the conjugation of monocrystalline iron oxide nanoparticles to anti–human E-selectin. In cell culture, umbilical vein endothelial cells avidly bind this MR contrast agent compared with controls when exposed to the inflammatory mediator IL-1β.
Imaging of VEGF receptors (VEGFRs) has been successfully performed in living murine hindlimb ischemia models using radiolabeled vascular endothelial growth factor-121 (VEGF121) and 64 Cu-VEGF121 PET. Microvessel density as measured with laser Doppler imaging correlated strongly with uptake of VEGF121 radiotracer. Imaging of integrin α V β 3 has been successfully performed using 18 F-labeled PEGylated tetrameric RGD peptide in tumor angiogenesis models. The application of such tracers to arthritis models may prove to be an important biomarker of aggressive forms of synovitis.
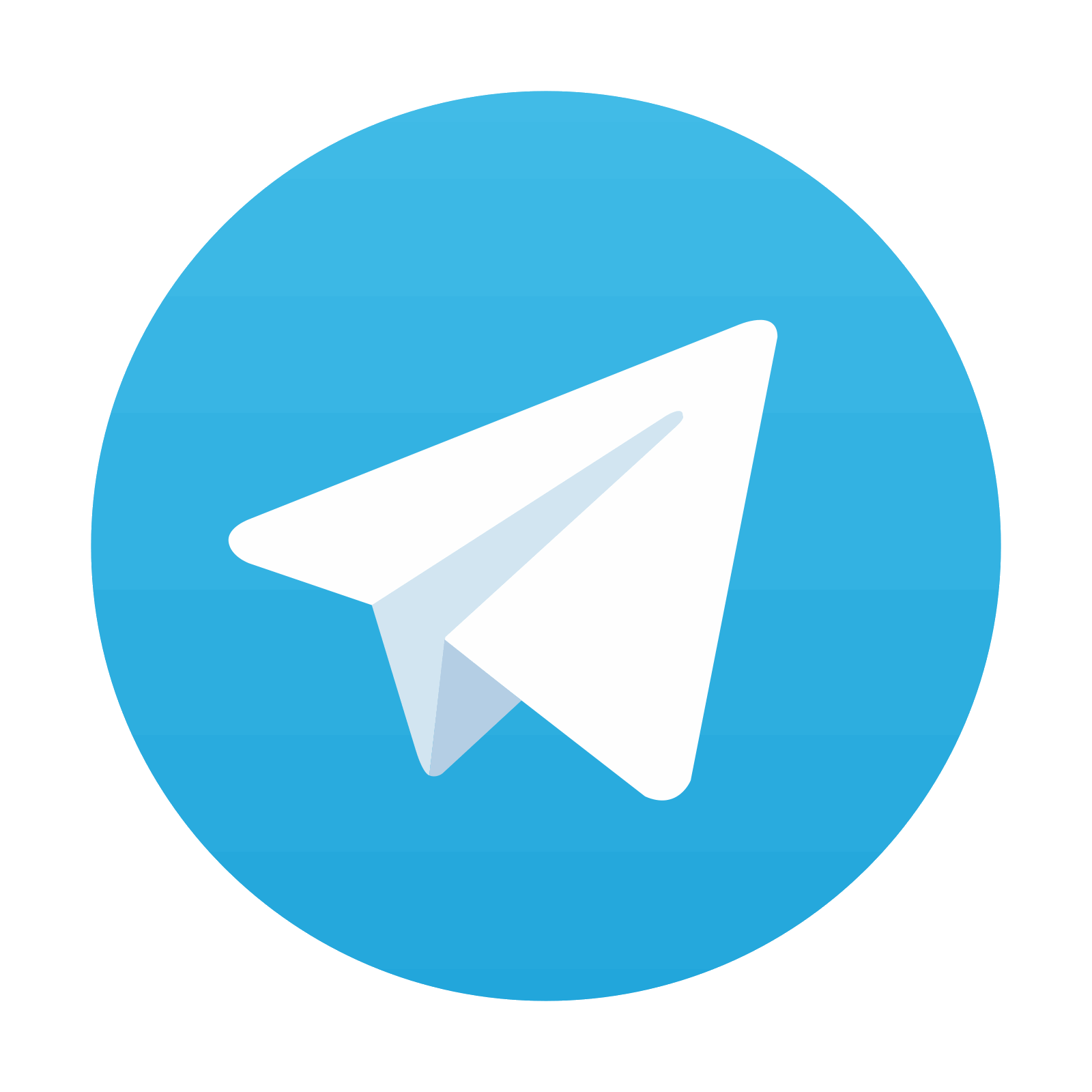
Stay updated, free articles. Join our Telegram channel
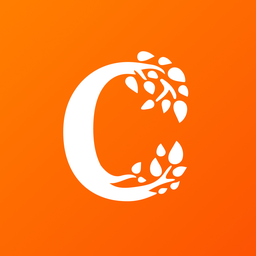
Full access? Get Clinical Tree
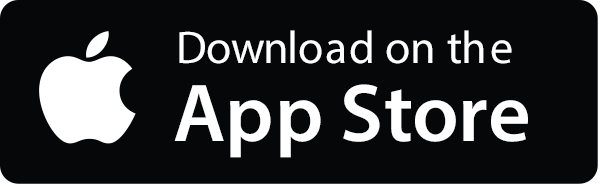
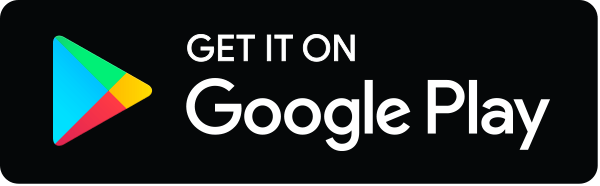