Meniscus and Labrum
Taylor J. Wiley, MD
David J. Tennent, MD, MAJ MC
Chathuraka T. Jayasuriya, PhD
John Twomey-Kozak, BS
Brett D. Owens, MD
Dr. Tennent serves as a board member, owner, officer, or committee member of the Society of Military Orthopaedic Surgeons. Dr. Owens or an immediate family member serves as a paid consultant to or is an employee of CONMED Linvatec, Flexion Therapeutics, Mitek, Musculoskeletal Transplant Foundation, and Vericel; has received research or institutional support from Hisogenics; and serves as a board member, owner, officer, or committee member of the American Orthopaedic Society for Sports Medicine. None of the following authors or any immediate family member has received anything of value from or has stock or stock options held in a commercial company or institution related directly or indirectly to the subject of this chapter: Dr. Wiley, Dr. Jayasuriya, and Dr. Twomey-Kozak.
Keywords: anatomy; labrum; meniscus
INTRODUCTION
The knee meniscus and glenoid labrum are anatomically distinct yet analogous structures. Although the gross anatomy differs, both tissues are composed of fibrocartilage with a complex but well-organized collagen microstructure. The meniscus and labrum function to increase congruity and stability, decrease contact stresses, and distribute load across their respective joints. This chapter will outline the embryologic development, anatomy, microstructure, and function of the meniscus and labrum.
MENISCUS
NORMAL EMBRYOLOGIC DEVELOPMENT
By 8 to 10 weeks of gestation, the menisci arise from a condensation of the intermediate layer of mesenchymal tissue to form their typical shape.1 The medial meniscus covers approximately 60% and the lateral meniscus 80% of the corresponding tibial plateau, and this proportion remains consistent throughout development.2 During development, the vascularity arising from the periphery initially extends throughout the meniscal width, but gradually decreases to supply only the peripheral 10% to 30% of the meniscus by 10 years of age.2 In addition, meniscal cellularity declines, whereas collagen content increases and organizes in a circumferential fashion.2
GROSS ANATOMY
The knee menisci are wedge-shaped fibrocartilaginous structures situated between the femoral condyles and tibial plateau. The macrostructure of the meniscus is divided into its tibial attachments at the anterior and posterior roots, the curved anterior and posterior horns, and the meniscal body at its midportion. The medial meniscus has a semicircular shape, which covers approximately 50% to 60% of the medial tibial plateau in adulthood.3 The posterior horn averages 11 mm in the anterior-posterior dimension, whereas the anterior horn is narrower.4 Figure 1, A illustrates the basic anatomy of the knee menisci and their attachments. Figure 1, B is a photograph diagram of a human tibial plateau with intact medial and lateral menisci. The anterior horn of the medial meniscus attaches to the tibia anterior to the anterior cruciate ligament (ACL) near the intercondylar fossa with significant variability. The transverse, or intermeniscal, ligament connects the anterior horns of the medial and lateral menisci (Figure 1, A). The posterior root attachment lies in the posterior intercondylar fossa between the lateral meniscus and posterior cruciate ligament.5 In addition to its root attachments, the meniscotibial (coronary) ligament stabilizes the medial meniscus through its attachment from the inferior aspect of the posterior horn to the tibia (Figure 1, A).
Peripherally, the medial meniscus is firmly attached to the deep medial collateral ligament and joint capsule, limiting its mobility.5
Peripherally, the medial meniscus is firmly attached to the deep medial collateral ligament and joint capsule, limiting its mobility.5
The lateral meniscus has a more circular C-shape with symmetric sizes of the anterior and posterior horns. The lateral meniscus anterior root attaches anterior to the intercondylar eminence and just lateral to the ACL insertion site on the tibia, whereas the posterior root attaches posterior to the lateral tibial spine just anterior to the medial meniscus posterior root.3 The popliteomeniscal fascicles extend from the lateral meniscus to the posterior capsule to create the popliteal hiatus. The meniscofemoral ligaments are variably present structures which connect the posterior horn of the lateral meniscus to the medial femoral condyle. These are described by their relationship to the posterior cruciate ligament, with the ligament of Humphrey crossing anterior to the PCL and the ligament of Wrisberg posteriorly. The less continuous attachment of the lateral meniscus to the capsule allows for greater meniscal mobility. Several studies have demonstrated this, including the work of Thompson et al, which reported a mean lateral meniscus excursion of 11.2 mm versus a mean medial meniscus excursion of 5.1 mm occurring from knee extension to flexion.6 Menisci have three zones that can be discerned based on vasculature and extracellular matrix composition (Figure 2). These zones are commonly described as white-white (ww), red-white (rw), and red-red (rr) zones (Figure 2).
VASCULAR ANATOMY
The blood supply of the menisci arises from the superior, middle, and inferior (medial and lateral) geniculate arteries as they branch from the popliteal artery. Synovial artery branches supply the anterior and posterior root attachments, whereas synovial and capsular artery branches form a perimeniscal capillary network to supply the peripheral 10% to 30% of the meniscus.7 From the anterior and posterior horns, endoligamentous vessels branch
into the substance of the menisci to perform terminal loops and provide direct nutrition over a short distance.8 The inner one-third of the meniscus is an avascular zone which receives its nutrition from the synovial fluid via loading diffusion.9
into the substance of the menisci to perform terminal loops and provide direct nutrition over a short distance.8 The inner one-third of the meniscus is an avascular zone which receives its nutrition from the synovial fluid via loading diffusion.9
NEURAL ANATOMY
The posterior articular branch of the tibial nerve innervates the knee along with contributions from the terminal branches of the obturator and femoral nerves. In addition, the recurrent peroneal branch of the common peroneal nerve supplies lateral portion of the capsule. Nerve fibers enter the capsule and peripheral portion of the menisci along a similar path as the blood supply. The highest nerve density lies in the anterior and posterior meniscal horns, whereas the peripheral third of the body has greater innervation than the middle third.10 Three types of mechanoreceptors have been identified in the human meniscus. Ruffini endings are type 1 mechanoreceptors, which have a low threshold and adapt slowly to pressure and joint deformation. Pacinian corpuscles (type II mechanoreceptors) have a low threshold but adapt quickly to alterations in tissue tension. Golgi tendon organs (type III mechanoreceptors) have a high signal threshold and exist in a greater concentration in the posterior horns, where they play a role in neuromuscular inhibition as the knee reaches terminal flexion.11
COMPOSITION AND MICROSTRUCTURE
The human meniscus is a well-organized structure consisting of fibrochondrocytes dispersed among a dense extracellular matrix. As the predominant meniscal cell type, fibrochondrocytes serve to produce and maintain the extracellular matrix. The fibrochondrocytes in the superficial layer of the meniscus appear similar to fibroblasts with their fusiform shape, whereas those in the deeper portion of the meniscus are more ovoid and chondrocyte-like.12 To assist in their matrix production function, fibrochondrocytes contain abundant endoplasmic reticulum and Golgi complexes, whereas a paucity of mitochondria suggests energy production occurs via anaerobic glycolysis.13
Water contained within the extracellular matrix accounts for 65% to 75% of the overall meniscal weight and volume.3 Composing 75% of the dry weight, collagens are the predominant extracellular matrix component and function to provide tensile strength to the meniscus.14 Type I collagen comprises the vast majority of this collagen content, with small contributions of collagen types II, III, V, and VI.13 Random collagen fiber orientation in the most superficial layer of the meniscus provides tensile strength and resistance to shear forces. In the deeper layers, the type I collagen fibers are organized to run circumferentially within the meniscus parallel to its peripheral border. The circumferentially oriented fibers are bound together by interspersed radially oriented tie fibers providing structural support.3 This organization allows for conversion of vertically oriented compressive forces into circumferential hoop stresses distributed throughout the meniscus.12
Matrix proteins other than collagen account for 8% to 13% of the meniscal dry weight.3 Fibronectin is a matrix protein involved in cellular processes such as cell migration/adhesion and tissue repair, whereas elastin likely contributes to tissue resiliency.13 Proteoglycans are large negatively charged molecules consisting one or more glycosaminoglycan side chains covalently bonded to a core protein. These hydrophilic molecules exist within the extracellular matrix and contribute to 1% to 2% of the dry weight, with the major type found in the meniscus being aggrecan followed by decorin, biglycan, and fibromodulin.12 Proteoglycans play an important role in the hydration of meniscal tissue and its viscoelastic ability to resist compressive forces. The meniscal horns and inner 50% of the weight-bearing meniscus contain the highest concentration of glycosaminoglycan side chains.15 The most common glycosaminoglycans in the adult meniscus include chondroitin-6-sulfate (40%), chondroitin-4-sulfate (10% to 20%), dermatan sulfate (20% to 30%), and keratan sulfate (15%).14,16
FUNCTION
Although previously believed to be a vestigial structure of the knee,17 the meniscus has multiple important biomechanical functions crucial to the normal function of the knee. The menisci increase the contact area and congruity, in turn decreasing contact stresses, across the tibiofemoral joint.18 Shock absorption, load transmission, stability, nutrition, joint lubrication, and proprioception are vital functions provided by the meniscus.3
The load-bearing function of the meniscus occurs as axial weight-bearing forces through the knee are converted to tensile forces along the circumferential collagen fibers of the meniscus.19 The firm anterior and posterior root attachments allow these hoop stresses to be absorbed without meniscal extrusion.20 Studies have demonstrated that 50% of the load through the medial compartment and 70% of the lateral compartment load transmission occurs through the menisci.21 The compressive loads through the posterior horns increase during knee flexion, from 50% in extension to 85% transmission in 90° flexion.18 Medial meniscectomy reduces the femoral condyle contact area by 50% to 70% and increases contact stress by 100%, whereas total lateral meniscectomy decreases contact area by 40% to 50% with a resultant increase in lateral compartment contact stress by 200% to 300% of normal.22,23 The normal knee demonstrates a shock absorbing capacity roughly 20% higher than previously meniscectomized knees.19 The clinical significance of these alterations was highlighted in a study by Pengas et al,24 which demonstrated a statistically significant increase in osteoarthritis and a 132-fold likelihood of total knee arthroplasty in a cohort of adolescent patients after total meniscectomy at a mean follow-up of 40 years.24
The menisci contribute to joint stability as the concave superior meniscal surface increases the congruity between the convex femoral condyles and flat tibial plateau. This stabilizing effect has been best demonstrated in ACL-deficient knees,
where medial meniscectomy has been reported to increase anterior-posterior tibial translation over 50% with the knee at 90° flexion.25 The posterior horn of the medial meniscus has been shown to be the most essential structure to resisting anterior tibial force in the ACL-deficient knee.26 The lateral meniscus acts as a secondary stabilizer against rotatory and valgus loads during pivot shift maneuvers in ACL-deficient knees.27
where medial meniscectomy has been reported to increase anterior-posterior tibial translation over 50% with the knee at 90° flexion.25 The posterior horn of the medial meniscus has been shown to be the most essential structure to resisting anterior tibial force in the ACL-deficient knee.26 The lateral meniscus acts as a secondary stabilizer against rotatory and valgus loads during pivot shift maneuvers in ACL-deficient knees.27
The menisci are thought to contribute to the nutrition and lubrication of the knee, although the mechanism for this has not been fully elucidated. Bird and Sweet used scanning electron microscopy to demonstrate a system of microcanals within the meniscus which communicated with the synovium.28 These canals have been postulated to provide fluid transport for nutrition and joint lubrication. The menisci may reduce frictional forces through compression of synovial fluid into the articular cartilage. As noted previously, mechanoreceptors exist within the anterior and posterior meniscal horns to provide joint proprioception. The rapidly adapting Pacinian corpuscles relay joint motion, whereas the slow-adapting Ruffini endings provide joint position sense.29
MENISCAL INJURY AND HEALING RESPONSE
Injuries to the meniscus that occur within the peripheral one-third exist within the vascularized portion of the meniscus (rr zone) and may undergo a healing response. After injury, an initial inflammatory response is quickly followed by formation of a fibrin clot. Undifferentiated mesenchymal stem cells migrate to the zone of injury from the vasculature. A new matrix is produced and healing occurs via the formation of fibrous scar tissue.30 The inflammatory response after meniscal injury has been investigated in multiple studies, with particular interest on its contribution to future osteoarthritis. In a rabbit model, Becker et al demonstrated increased expression of vascular endothelial growth factor (VEGF) for angiogenesis after meniscal injury.31 Brophy et al found an increased catabolic response to meniscal injury, with elevation of several osteoarthritis-related markers including proinflammatory cytokine interleukin 1 beta (IL-1β) and several matrix metalloproteinases occurring to a greater extent in patients younger than 40 years.32 Carter et al demonstrated increased matrix metalloproteinase activity in synovial fluid reflective of greater cartilage strain after meniscal injury.33
With regard to the time line for meniscal healing, a dog model previously demonstrated meniscal repair tissue attaining 80% of original strength at 12 weeks.34 Meniscal injuries within the inner one-third, or ww zone, and within the central one-third, or rw zone, have limited capacity for healing given the avascularity of the tissue. Given this poor healing response, a multitude of research has been directed toward biologic adjuvants for meniscal repair. The earliest adjuvant was synovial abrasion with a meniscal rasp to increase vascular response, as well as trephination of the peripheral meniscus and capsule.30 Because of the improved meniscal healing rates in the setting of concomitant ACL reconstruction, bone marrow stimulation techniques have been used to release bone marrow-derived mesenchymal cells in the setting of meniscal repair.35 The direct application of platelet-rich plasma and/or pluripotent stem cells has been investigated in animal and human studies with variable results.36,37,38 Several stem/progenitor cell sources are actively being investigated for their therapeutic healing effects that have been observed in animal models and tissue explant models of meniscus injury repair (Table 1). Cell sources include mesenchymal progenitor/stem cells from adipose, synovium, cartilage, and bone marrow. Cell-based therapies are promising and they are being used both by themselves and in combination with growth factors and scaffolds in preclinical settings to optimize efficacy.
LABRUM
ANATOMY
DEVELOPMENT
During normal fetal development, the superior glenoid labrum begins to coalesce with the biceps tendon by approximately 9 weeks of gestation. Further anterior and posterior consolidations appear and transition to a highly vascular, primitive fibrocartilaginous structure with distinct transitions from glenoid to labrum from approximately 12 to 20 weeks.39,40 However, mature labral tissue is not present until full term with a gradual transition to mature fibrocartilage over the first several years of life.40,41 Throughout life, an increased proportion of elastin and decreased vascularity has been found to correlate with increasing age.41
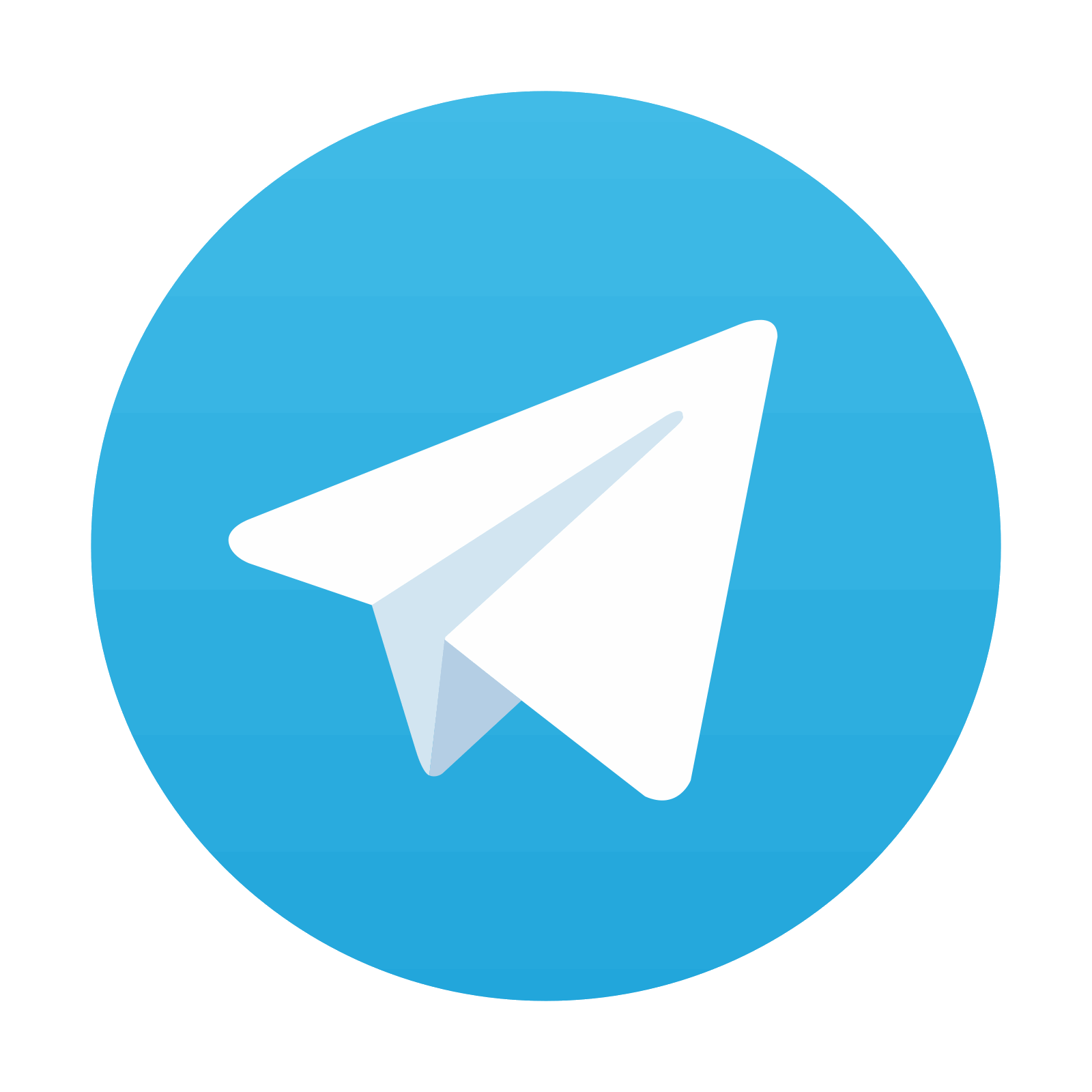
Stay updated, free articles. Join our Telegram channel
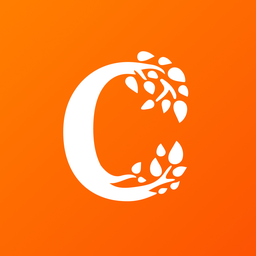
Full access? Get Clinical Tree
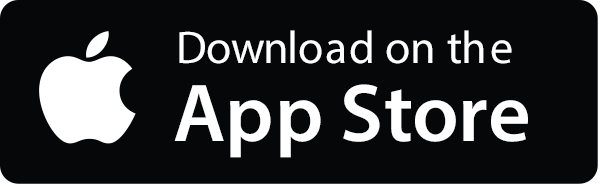
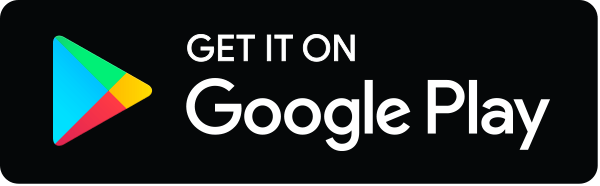
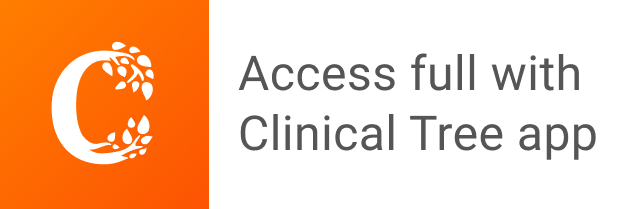