Abstract
For a few years, the non-invasive modulation of motor cortex has become the centre of much attention because of its possible clinical impact. Among the different mechanism allowing to modify motor-cortex excitability, transcranial direct current stimulation (tDCS), with its efficacy and ease of use, plays a major role. The aim of this review is to improve the understanding of the underlying mechanisms of the tDCS effect in the field of rehabilitation. The mechanisms underlying tDCS effects when applied over the motor cortex differ depending on the polarity used. Moreover, the mechanisms underlying these effects differ during stimulation (per-stimulation) and after the end of it (after-effects). This review highlights the known mechanisms involved in tDCS effects on brain excitability and illustrates that most remain not well understood and debated. Further studies are necessary to elucidate the mode of action of tDCS and determine the best paradigm of stimulation depending on the goals.
1
Introduction
In the past few years, the non-invasive modulation of the motor cortex has generated much attention in light of its possible clinical impact. Among the different mechanisms allowing to modify motor-cortex excitability, 2 tools play a key role: repetitive transcranial magnetic stimulation (rTMS) and transcranial direct current stimulation (tDCS). With rTMS, the large rapidly changing magnetic field of a pulse induces an electrical stimulating current in the brain able to generate action potentials in the cortex and white matter. With tDCS, a portion of the long-lasting applied current enters the skull and modulates brain excitability without generating action potential.
However, the main mechanisms underlying the effects of the 2 tools differ. This article aims to improve our understanding of the underlying mechanisms of the tDCS effect in rehabilitation. We highlight the different mechanisms responsible for the effect observed during stimulation and after the end of stimulation (after-effects). This explanation will allow for a better understanding of why the tDCS effects are polarity-dependent. Finally, we show that these mechanisms remain partly unexplained and therefore further studies are required before this tool can be used in the clinic to decrease impairments in patients with central nervous system lesions.
2
Methodological point
We searched MEDLINE via PubMed with the following keywords: “transcranial direct current stimulation” and “mechanisms” and identified 274 references. However, when “rehabilitation” was associated with tDCS, the number of references increased to 352. Therefore, we limited our research to combining “tDCS” with “mechanism”; 45 references were chosen for their pertinence to this review.
2
Methodological point
We searched MEDLINE via PubMed with the following keywords: “transcranial direct current stimulation” and “mechanisms” and identified 274 references. However, when “rehabilitation” was associated with tDCS, the number of references increased to 352. Therefore, we limited our research to combining “tDCS” with “mechanism”; 45 references were chosen for their pertinence to this review.
3
Mechanisms underlying tDCS effects
Systematic investigations of the behavioural effects induced by direct current (DC) stimulation to the scalp in normal subjects date back at least 30 to 40 years. Using a very low scalp DC up to 50–500 μA in 32 healthy subjects, Lippold and Redfean (1964) found that scalp anodal currents increased alertness, mood and motor activity, whereas cathodal polarization produced quietness and apathy. DC passed through 2 frontal electrodes and 1 over the right knee . Using a double-blind experimental design with objective methods for estimating mood and alertness in 6 healthy subjects, Scheffield and Mowbray (1968) failed to confirm the previous findings and concluded that scalp DC had no significant effect . Similarly, Hall et al. (1970), studying 18 normal subjects with a double-blind experimental design, reported that currents up to 0.3 mA left the reaction time unchanged after an acoustic “go” signal . More recently, in the late 1980s, Jaeger et al. observed that weak scalp DC (0.3 mA) affected the reaction time to an acoustic stimulus and the choice of the hand to push a button in response to an acoustic signal. These findings in normal subjects show that DC stimulation to the scalp may induce an important variety of excitability changes at the cortical level .
The first modern study demonstrating the modification of cortical excitability induced by tDCS was by Priori et al., in 1998 . The authors tested the effects of tDCS on the excitability of the cerebral cortex using transcranial magnetic stimulation (TMS; a technique able to test cortical motor-area excitability directly), thus overcoming interpretative problems arising from previous phenomenological and descriptive studies. In 4 different experiments, they tested the functional effects of very weak DC stimulation (< 0.5 mA, duration < 7 s) on the motor areas of human cerebral cortex by studying changes in motor-evoked potential (MEP) elicited in small hand muscles in 15 healthy subjects. The authors placed the 2 electrodes over the skull and, with TMS, induced the motor response just before the end of the tDCS sequence. Anodal tDCS slightly but significantly and consistently reduced (by some 8%) the size of the controlled unconditioned motor response, whereas cathodal tDCS left the response unchanged. The authors also found that higher anodal DC stimulation produced progressively stronger depression of MEP. These findings provided the first direct evidence that a very small electrical field crosses the skull and affects brain excitability .
These initial findings were partially confirmed by the study of Nitsche and Paulus, in 2000, which is now considered the reference for tDCS ( Fig. 1 ). In healthy subjects, the authors showed too that the modifications of the motor-cortex excitability induced by tDCS were polarity-dependent. They showed for the first time in humans, using a TMS approach to assess motor-cortex excitability, that anodal tDCS increases motor-cortex excitability, whereas cathodal tDCS decreases it. Indeed, anodal tDCS increased MEP amplitude by about 40%, whereas cathodal tDCS decreased it in the same range. The authors demonstrated that tDCS could modulate brain excitability and that:
- •
the best configuration to modify motor-cortex excitability was to place the active electrode in regards of the motor cortex and the other electrode on the contralateral supra orbital region;
- •
the effects of tDCS were present during stimulation and could last after the end of stimulation (“after-effects”) ( Fig. 2 );
Fig. 2
Polarity-specific after-effects of direct current stimulation. Time course of polarity-specific motor-cortex excitability changes outlasting stimulation duration, shown after 5 min of direct current stimulation at 1 mA. MEP amplitudes returned to baseline within 5 min. Asterisks indicate significant differences between MEP amplitudes after stimulation and at baseline (two-tailed t test, paired samples, P < 0.05).
Figure from Nitsche and Paulus .
- •
the importance of the motor-cortex excitability changes depended on the intensity of stimulation;
- •
and at least 3 min of tDCS at 1 mA or an intensity of 0.6 mA for 5 min was necessary to induce after-effects .
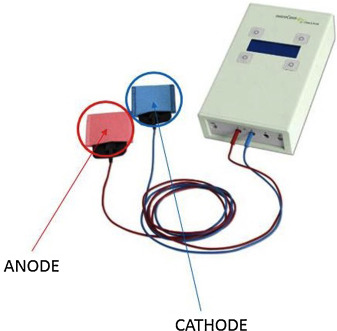
Since this study, all the following studies that assessed the impact of tDCS on the motor cortex used the same design, with the same placement of electrode and duration of tDCS stimulation most of the time between 10 and 20 min at 1 to 2 mA. Since this time, this technique appears thus as a promising tool to modulate motor-cortex excitability and to induce either long-term potentiation or long-term depression.
The following studies tried to better define the main mechanisms underlying the polarity-dependent effects induced by tDCS. The results of these studies detailed as follows suggest that the mechanisms involved during stimulation differ from those during the after-effects.
3.1
Mechanisms underlying tDCS effects during stimulation
The effects of weak polarizing currents appear to critically depend on both the strength of the current applied and the duration of the application. DC stimulation is often described in terms of the charge density (C/cm 2 ), where 1 Coulomb (C) is the amount of the electric charge transported in 1 s by a steady current of 1 A.
Early studies in animals using direct cortical stimulation with a stimulus of 0.00013 to 0.3 C/cm 2 showed that if the anode was placed above the cortex, spontaneous neuronal activity was increased, whereas cathodal polarity resulted in reduced spontaneous discharges due to sub-threshold changes in membrane polarisation . However neurons throughout the cortex were not modulated in a homogenous manner. Neurons in deep cortical layers were often deactivated by anodal stimulation and activated by cathodal stimulation . This finding would suggest that the orientation of neurons relative to the electrical fields is of vital importance to their response to stimulation. In addition, the different subpopulations of neurons appear to have different thresholds of modulation. For example, non-pyramidal tract neurons were stimulated at lower total charges than pyramidal tract neurons, the activity of which was modulated only at charges > 0.0008 μC/cm 2 . These findings are important for human studies because they suggest that depending on the paradigm of stimulation used, tDCS is able to stimulate both pyramidal tract neurons and interneurons.
Results from humans suggest that the effects of tDCS during stimulation appear to depend solely on changes in membrane potentials. Indeed, Nitsche et al., using a pharmacological approach, found important results supporting this hypothesis. The authors assessed the impact of a sodium-channel blocker (carbamazepine) and a calcium-channel blocker (flunarizine) on tDCS-elicited motor cortical excitability changes in healthy human subjects . Blocking voltage-dependent sodium channels completely eliminated the enhanced excitability observed during anodal tDCS and blocking the calcium channel diminished it. These results agreed with those observed in animals by Purpura and McMurtry, in 1965 , who also observed that reduced excitability induced by cathodal tDCS was not changed by ion channel blockade. Because the activity of both channels is voltage-dependent , this result could be due to cathodal tDCS-generated neuronal hyperpolarization, which in animals may represent the main mode of action of this type of tDCS stimulation . This last result also indicates that further investigation is necessary to better understand the main mechanisms involved in the hyperpolarization induced by cathodal stimulation.
Moreover, concerning anodal stimulation, neither dextromorphane (antagonist of N-methyl-D-aspartate [NMDA] receptor) nor lorglumide (LOR; a gamma aminobutyric acid A [GABA A ] receptor agonist) had a modulatory effect on the per-stimulation response . Anodal tDCS did not alter TMS measures of glutamatergic interneurons (absence of modification of intracortical facilitation) or gabarergic interneurons (absence of modification of short interval cortical inhibition). Thus, no significant modulation of the excitability of either glutamatergic or gabaergic internerneurons occurred during tDCS .
A recent publication by Bikson et al. suggested new types of reflexions to understand the other possible mechanisms induced by tDCS during stimulation . Indeed these authors suggest that tDCS action could be more accurately described as redistributing polarization across the cellular axis, for example, one dendritic branch versus another . This change in weight across the dendrite may provide a cellular substrate to affect the input bias of a network. The authors also suggested that polarization of the afferent axon itself appears to activate a specific pathway .
3.2
Mechanisms underlying tDCS after-effects
Concerning the possible mechanisms involved in the after-effects observed after tDCS sessions, a few hypotheses have been evoked.
With a pharmacological approach, the after-effects of anodal tDCS depend on membrane polarization. Indeed the administration of a calcium-or sodium-channel blocker abolished the after-effects of tDCS . In addition, Liebantz et al. showed that dextromorphane (antagonist of NMDA receptor) prevented the induction of long-lasting after-effects induced by tDCS, whatever the polarity used. These results likely suggest that after-effects induced by tDCS rely on modification of NMDA-receptor sensitivity. Dopaminergic receptors participate in NMDA-receptor-dependent neuroplasticity . Nitsche et al. showed that D2 receptor blockage by sulpiride abolished the induction of the after-effects by tDCS. This result supports the NMDA receptor playing a role in the after-effects observed after a tDCS session .
Another explanation relies on the modification of intracortical neurotransmitter concentrations. Indeed, Stagg et al., using magnetic resonance spectroscopy, assessed the polarity-sensitive modulation of cortical neurotransmitters induced by tDCS . After anodal stimulation, the GABA concentration decreased significantly by 9.2 ± 5.3% relative to sham stimulation, with no change in glutamate concentration. In contrast, after cathodal tDCS, the glutamate concentration decreased by 19.1 ± 5.3% as compared with sham stimulation and the GABA concentration decreased by 11.1 ± 6.8%. The reduction in GABA and glutamate concentrations was positively correlated. Therefore, the modification in NMDA-receptor sensitivity seems not the only mechanism responsible for the tDCS after-effects whatever the polarity used and the GABA neurotransmitter may play a key role in these tDCS-induced after-effects ( Fig. 3 ).
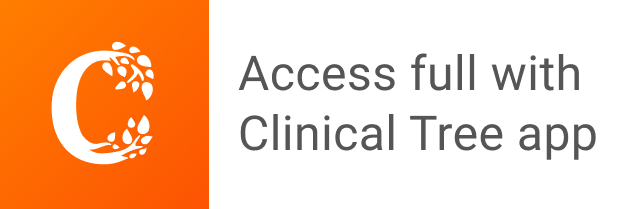