Fig. 10.1
Proposed model of exercise-induced signaling pathway in the skeletal muscle. Classified into three signaling pathways: AMP-activated protein kinase (AMPK), Ca2+, and mitogen-activated protein kinase (MAPK). AMPK is activated by detection of an increased intracellular AMP/ATP ratio. LKB1 and Ca2+/calmodulin kinase kinase (CAMKK) are also known as upstream kinases of AMPK. AMPK serves as a metabolic regulator, which activates and/or inactivates the downstream molecules related to metabolic regulation. Ca2+ activates Ca2+/calmodulin-dependent protein kinase (CaMK), calcineurin, and protein kinase C (PKC). These signaling pathways regulate transcriptional factors and regulate glucose metabolism and fiber type transition. The MAPK signaling pathway including p38, c-jun NH2-terminal kinases (JNK), and extracellular signal-regulated kinase (ERK) is also activated by muscle contraction. MAPK mainly regulates downstream transcription factors and coactivators, which results in myogenesis and muscle adaptation
When skeletal muscles start contraction, intracellular energy levels are dynamically changed. Muscle contraction accelerates ATP consumption and increases AMP. Namely, the intracellular AMP/ATP ratio is increased, which results in the activation of AMP-activated protein kinase (AMPK). AMPK serves as a metabolic regulator, which activates and/or inactivates the different kinds of signaling proteins related to glucose, fatty acid, and protein metabolism (Fig. 10.1).
The mitogen-activated protein kinase (MAPK) signaling pathway, including p38, c-jun NH2-terminal kinases (JNK), and extracellular signal-regulated kinase (ERK), is known to transmit environmental changes into intracellular responses. MAPK is ubiquitously expressed and regulates gene transcriptions and metabolisms. Exercise/contraction also activates the MAPK signaling pathway, which regulates gene expressions and metabolism (Fig. 10.1). Understanding these signaling pathways and resultant metabolic regulations may contribute toward explaining the beneficial effects of exercise.
10.2 Ca2+ Signaling Pathway Induced by Muscle Contraction
Ca2+ is the first trigger to induce muscle contraction. The action potential traverses the T-system [1] and reaches the sarcoplasmic reticulum to release Ca2+. An increase in sarcoplasmic Ca2+ plays a pivotal role to induce not only excitation–contraction coupling but also serves as the regulator for many cellular events via several pathways including the activation of CaMK, calcineurin, and PKC [2].
CaMK is a serine/threonine protein kinase which is classified into the following two categories: kinases with a single substrate such as phosphorylase kinase, CaMKIII, and myosin light chain kinase, and multifunctional kinases with several substrates such as CaMKI, CaMKII, and CaMKIV [3]. Of those, CaMKII is of particular interest in the skeletal muscle. CaMKII has four isoforms (α, β, γ, and δ) and each isoform is encoded by separate genes [3]. CaMKII consists of 8–12 similar homologous subunits held together by interactions between a C-terminal association domain and forms a pinwheel-like structure [3]. When one subunit is activated by binding a Ca2+–calmodulin complex, it phosphorylates the other subunits in the same enzyme (inter-subunit autophosphorylation). This complex system results in the delayed regulation of CaMKII activity in response to elevated Ca2+. Therefore, CaMKII is considered to act as the stimulation–frequency decoder in the skeletal muscle, which decodes the frequency of Ca2+ spikes into graded amounts of kinase activity [4].
CaMKII activation induced by acute exercise enhances glucose transport. In mice, overexpression of the specific inhibitor of CaMKII in the skeletal muscle decreased glucose transport induced by muscle contraction without affecting AMPK phosphorylation. This suggests that CaMKII has a critical role in the regulation of glucose uptake independent of the AMPK pathway [5]. CaMK also affects gene expression levels related to glucose transport and mitochondrial biogenesis. Myocyte enhancer factor-2 (MEF2), a transcription factor, and class II histone deacetylases (HDACs) are the key factors for regulating gene expressions related to glucose transport [6–8]. In the basal state, a complex of HDACs and MEF2 bind to the MEF2 target genes and deacetylates histones surrounding the MEF2 binding domain and represses the transcription of target genes [9]. When activated, CaMKII phosphorylates HDACs. Then, MEF2 is released from the phosphorylated HDACs and exported to the cytoplasm. The released MEF2 is able to associate with coactivators such as p300 and PGC-1α, which induce transcription [10, 11]. In rats, swimming exercises increased MEF2 binding to the glucose transport type 4 (Glut4) promoter, a critical glucose transporter in the skeletal muscle, and in the acetylation of histone H3 on the Glut4 gene [12]. Rats injected with KN-93, an inhibitor of CaMK, attenuated the MEF2 binding, histone acetylation, and Glut4 expression [12]. This suggests that CaMKII activation is important to increase Glut4 expression.
Calcineurin is a Ca2+-dependent serine/threonine phosphatase which is the heterodimer of a catalytic unit, calcineurin A (α, β, or γ), and a regulatory subunit, calcineurin B (1 or 2). In the skeletal muscle, calcineurin A-α, calcineurin A-β, and calcineurin B1 are expressed [13]. Calcineurin is activated by calmodulin [14], which is related to muscle hypertrophy [15, 16] and muscle fiber transition [17, 18]. Calcineurin-deficient mice showed reduced type I fibers [19]. Mice overexpressing muscle-specific calcineurin showed an increase in type I fibers and mitochondrial oxidative function [17, 18], suggesting that calcineurin regulates type I fiber transition. In addition, calcineurin activated the myosin heavy chain (MHC) IIa promoter rather than the MHCIIb promoter [20, 21], suggesting that calcineurin also serves as the fiber type transition within the type II fiber. Two transcription factors, nuclear factor of activated T-cells (NFAT) and MEF2, are the downstream targets of calcineurin in muscle fiber type regulation. Calcineurin directly binds to dephosphorylate NFAT. Then NFAT is translocated to the nucleus and regulates gene expression including myf5, myoglobin, MHC, and troponin [22]. Calcineurin also stimulated the transcriptional activation function of MEF2 in the skeletal muscle using transgenic mice and cultured myocytes [6], and cyclosporin A, a calcineurin inhibitor, blocked this effect [23]. These studies suggest MEF2 and NFAT serve as important transcriptional factors in the fiber type transition. On the other hand, whether calcineurin is related to muscle hypertrophy is still controversial. With reference to many genetically modified mice models, some have reported that calcineurin is not related to muscle size [17, 19, 24], while others showed calcineurin was related to muscle size [15, 16]. There is a relationship between fiber type transition and muscle size as the type I fiber is resistant to muscle atrophy in comparison to the type II fiber [25]. Therefore, to determine whether calcineurin contributes to hypertrophy, a comprehensive understanding of the mechanism, including fiber type transition and atrophy, is needed.
PKC is classified into three groups. The conventional PKC (cPKCs, α, β1, β2, and γ isoforms) is directly activated by Ca2+[26], the novel PKCs (nPKCs, δ, ε, θ, and η isoforms) are dependent on only diacylglycerol for activation, and the atypical PKCs (aPKCs, ζ, and λ isoforms) are activated independent of Ca2+ and diacylglycerol. cPKC activation is regulated by the frequency of Ca2+ spikes and the temporal coordination between Ca2+ spikes and diacylglycerol signals in rat basophilic leukemia 2H3 cells [27], although how this applies to the skeletal muscle still remains unclear. In early reports, total PKC was activated by muscle contraction in the rat skeletal muscle [28, 29]. However, recent studies suggested that isoform-specific analyses of cPKC and nPKC were not affected by exercise in humans [30, 31]. In contrast, aPKCs are reported to be activated by exercise in rats and humans [30–33]. In rat muscle and L6 myotubes, aPKCs were also activated by 5-aminoimidazole-4-carboxamide-1-β-D-riboside (AICAR), an activator of AMPK, and specific aPKC inhibitors blocked glucose uptake stimulated by AICAR. This suggests that glucose uptake via the AMPK pathway is mediated via aPKCs. Although some studies suggest that the effect of aPKC on glucose uptake was mediated by ERK signaling [32, 33], exercise-stimulated glucose uptake was not related to ERK signaling ([34] as described below). Therefore further studies to elucidate the effect of aPKC on glucose uptake will be required.
10.3 AMPK Signaling Pathway Induced by Muscle Contraction
Exercise/muscle contraction alters the energy status in the skeletal muscle. During exercise, AMPK senses an increased AMP level and decreased ATP level (an increase in the intracellular AMP/ATP ratio), and then AMPK is phosphorylated at the Thr172 site and regulates downstream molecules resulting in an increase in glucose uptake and fatty acid oxidation. Therefore, AMPK is considered as a fuel gauge regulating energy metabolism in the cells [35].
AMPK is a serine/threonine kinase that consists of a catalytic subunit (α-subunit) and regulatory subunit (β-subunit and γ-subunit). Each subunit has some isoforms: α-subunit (α1 and α2), β-subunit (β1 and β2), and γ-subunit (γ1, γ2, and γ3). Although the skeletal muscle expresses all isoforms, the major isoform is the heterodimer complex of α2β2γ1, α1β2 γ1, and α2 β2 γ3 in the human vastus lateralis muscle [36, 37]. The γ-subunit of AMPK has four cystathionine beta synthase (CBS) domains, which bind either AMP or ATP. When cellular energy status is low, such as from prolonged fasting or exercise, AMP binds to the CBS domain of γ-subunit. This results in a conformational change, activating AMPK, which turns on the ATP regenerative pathway and inhibits the ATP-consuming pathway.
In addition to detecting the energy status change, upstream molecules of the tumor suppressor protein kinase LKB1 [38] and Ca2+/calmodulin kinase kinase (CaMKK) [39] also phosphorylate Thr172 in AMPK and regulate AMPK activity. Muscle-specific LKB1 knockout mice showed a significant decrease in AMPKα2 activity under basal and contractive stimulation [40, 41]. LKB1 is a constitutively active protein and is not rate limiting for activation of AMPK [42]. When AMPK undergoes a conformational change induced by AMP binding to a CBS domain, LKB1 can easily access AMPK and serves to accelerate AMPK phosphorylation. Unlike AMPKα2, AMPKα1 activity is not affected by LKB1 knockout mice, suggesting that LKB1 is the predominantly upstream kinase of AMPKα2.
Another upstream kinase is CaMKK. CaMKKII is considered to be a dominant upstream of AMPK [39, 43]. In endothelial cells, activated AMPK via CaMKKII was dependent on thrombin but independent of LKB1 [44]. In T lymphocytes, T-cell antigen receptor stimulus activates AMPK via the CaMKK-dependent pathway without change of energy status [45]. In the skeletal muscle, CaMKK inhibitors of KN-93 and STO-609 reduced AMPK phosphorylation and activity, while the inhibitors did not affect the AICAR-stimulated glucose uptake or LKB1 activity [46]. This suggests that CaMKK regulates AMPK phosphorylation and glucose uptake independent of energy status and LKB1 pathway.
AMPK has been of considerable interest with respect to exercise-induced glucose uptake. Exercise/contraction induced activation of AMPK in humans [47, 48] and rodents [49, 50] and increased glucose uptake independent of the insulin pathway. Interestingly, genetically modified mice with an ablation of AMPK activity showed a normal contraction-induced glucose uptake [51, 52]. On the other hand, contraction-induced glucose uptake was inhibited in LKB1 knockout mice [40, 41], suggesting that other downstream molecules of LKB1 besides AMPK are related to contraction-induced glucose uptake. A recent study suggests that sucrose nonfermenting AMPK-related kinase (SNARK) is another substrate of LKB1 for regulating glucose transport [53]. As downstream molecules of AMPK, GTPase-activating proteins of the TBC1 domain family, members 4 and 1 [TBC1D4 (AS160) and TBC1D1] have been reported (see the review [54]). Activated AMPK by muscle contraction phosphorylates TBC1D4/TBC1D, which enhances Glut4 translocation and an increase in glucose uptake.
Activation of AMPK also promotes fatty acid metabolism. Carnitine acyltransferase 1 (CPT1) is a mitochondrial fatty acid transporter, which is allosterically inhibited by the malonyl-CoA. Malonyl-CoA is synthesized from acetyl-CoA catalyzed by acetyl-CoA carboxylase (ACC). AMPK activation inhibits ACC activity via an increase in ACC phosphorylation, which therefore decreases malonyl-CoA synthesis, resulting in CPT1 activation and promotes fatty acid uptake into mitochondria and an increase in fatty acid oxidation. Although AMPK plays a role in ACC phosphorylation, the necessity of AMPK in fatty acid oxidation in the skeletal muscle remains unclear. Mice with skeletal muscle-specific AMPKα2-deficient and AMPKα1-dominant-negative transgenes showed increased fatty acid oxidation when stimulated by in vivo exercise and isolated muscle contraction [55]. It was also reported that ACC is not the only regulator of fatty acid oxidation [55]. Therefore, fatty acid activation stimulated by exercise is not fully explained with an inactivation of ACC by AMPK activation. Some redundant pathway may exist for fatty acid oxidation induced by contraction.
Activated AMPK regulates not only downstream phosphoproteins related to metabolism but also gene expressions via transcription factors and a transcriptional coactivator [56]. PGC-1α is one of the transcriptional coactivators that interacts with the transcription factors and regulates many genes of energy homeostasis related to fat oxidation and mitochondrial biogenesis [57]. Activated AMPK directly phosphorylates PGC-1α at thr177 and ser538 [57]. Phosphorylation of PGC-1α regulates the gene expression of glut4, mitochondrial genes such as cytochrome C, ucp1/2, and even pgc-1 itself. AMPK also affects the transcription factors. Activated AMPK enhances the nuclear respiratory factor-1 (NRF-1) binding activity to its promoter, which enhances mitochondrial biogenesis [58]. AMPK directly phosphorylates and activates glut4 enhancer factor, which recruits the MEF2 and enhances glut4 transcription [59]. These data suggest that AMPK indirectly regulates gene expression via enhancement of coactivator activity and transcriptional factors. It is also reported that AMPK is related to fiber type transition. Continuous dosing of AICAR in rats showed a decreased ratio of type IIb fibers and an increased ratio of type IIx fibers in the extensor digitorum longus muscle [60]. AMPKγ1 transgenic mice with a chronic activation of AMPK showed an increase in type IIa/x fiber [61]. As a downstream molecule related to fiber type transition, PGC-1α is known to regulate fast-to-slow fiber type transformation [62]. However, AMPKα2 knockout mice [63] and dominant-negative mice [61] showed an increase in exercise-induced PGC-1α gene expression in the skeletal muscle with only a little impairment in the exercise-induced fiber transition. Therefore, it is not well understood how AMPK regulates fiber type transition.
10.4 The Mitogen-Activated Protein Kinase Signaling Pathway Induced by Muscle Contraction
MAPK signaling cascades play important roles in various kinds of cells, which are stimulated by environmental stressors, cytokines, and growth factors. Activation of the MAPK signaling pathway phosphorylates downstream substrates and finally transmits the transcription factors and coactivators. This results in the regulation of physiological processes such as cell proliferation, cell differentiation, apoptosis, inflammation, hypertrophy, energy metabolism, and gene transcription [64]. In the skeletal muscle, the MAPK signaling pathway such as the ERK1/ERK2, p38, and JNK is rapidly induced by muscle contraction [65].
10.4.1 p38 MAPK
Four isoforms of p38 (p38α, p38β, p38γ, and p38δ) were reported. p38α and p38β were ubiquitously expressed, while p38γ is expressed mainly in the skeletal muscle and p38δ is expressed mainly in the lung and kidney [66]. Muscle contraction increases p38 phosphorylation in rodents [67, 68] and humans [69, 70]. In an early study, activation of p38 MAPK by muscle contraction was thought to regulate glucose metabolism, since SB203580, a p38α/β antagonist, inhibited glucose uptake stimulated by AICAR [71]. However, later studies suggested that overexpression of p38γ in the skeletal muscle negatively regulates Glut4 expression and contraction-mediated glucose uptake [72]. In another report, overexpression of dominant-negative p38α/β did not affect insulin-stimulated glucose uptake [73]. Therefore, inhibition of SB203580 in the early study is considered to come from the direct inhibition of the glucose transporter [74]. It seems that activation of p38 induced by muscle contraction is not directly related to glucose transport in the skeletal muscle.
Activation of p38 by muscle contraction induced PGC-1α expression via the activation of transcription factor 2 (ATF2), a transcription factor of PGC-1α in C2C12 cells [75], and is related to the muscle adaptation. Skeletal muscle-specific expression of MKK6E, a constitutively active activator of p38, increased PGC-1α and cytochrome c oxidase IV (COX IV) protein expression in fast type skeletal muscles [75]. Muscle-specific ablation of p38γ, but not p38α and p38β, reduced endurance exercise-induced pgc-1α mRNA expression and mitochondrial proteins such as COX IV and cytochrome c [76]. p38 activation is also involved in myogenic differentiation. Using the primary myoblasts from the skeletal muscle of neonatal mice deficient in p38α, p38β, p38γ, or p38δ, p38α plays a critical role in myogenesis and p38γ plays a role in cellular fusion [77]. Although the relative contribution of the p38 subtypes in response to physiological conditions such as contraction and proliferation still remains unclear, at least p38 seems to contribute to myogenesis.
10.4.2 c-jun NH2-Terminal Kinases
JNK, members of the stress-activated protein kinase family, are encoded by three genes, juk1, juk2, and juk3 and produce ten isoforms via alternative splicing [78]. Transcripts derived from three juk genes produce 46 kDa (JNK1) and 55 kDa (JNK2) isoforms [78], although the functional difference of two splice variants remains unclear. JNK activity is related to insulin resistance [79, 80]. JNK1 knockout mice were protected from obesity-induced insulin resistance [81]. These JNK KO mice that were fed a high-fat diet had a significantly lower blood glucose level and decreased adipose size than the control mice [81]. In the muscle-specific JNK KO mice study [82], the mice showed improved high fat-induced insulin resistance. However, these mice did not influence diet-induced obesity. In particular, adipose tissue-specific JNK1 KO also showed improved insulin sensitivity with the diet-induced obesity phenotype [83], while liver-specific JNK KO mice did not [84]. In the skeletal muscle, muscle contraction and physical exercise increase JNK activity in the rat and human skeletal muscle [65, 70, 85–87]. Considering that the JNK KO mice showed improved insulin resistance, the activation of JNK by muscle contraction is confusing. One interpretation is that JNK in the skeletal muscle is not directly related to glucose metabolism but regulates other events via regulation of gene expression. The glycogen content and glycogen synthase activity stimulated by contraction was not different between control and skeletal muscle-specific JNK overexpressed muscles [88]. In addition, glucose uptake stimulated by the insulin or muscle contraction was not significantly different between control and JNK KO muscles [88], suggesting that JNK activity is not related to glucose metabolism in the skeletal muscle. JNK activation induced by muscle contraction is associated with an increased mRNA level of c-Jun, a downstream molecule of JNK in humans and rats [87, 89]. c-Jun is known as the transcription factor which regulates various cell events including cellular proliferation, apoptosis, DNA repair, and inflammation [90–92]. Therefore, JNK activation stimulated by muscle contraction may contribute to these events via c-jun.
< div class='tao-gold-member'>
Only gold members can continue reading. Log In or Register a > to continue
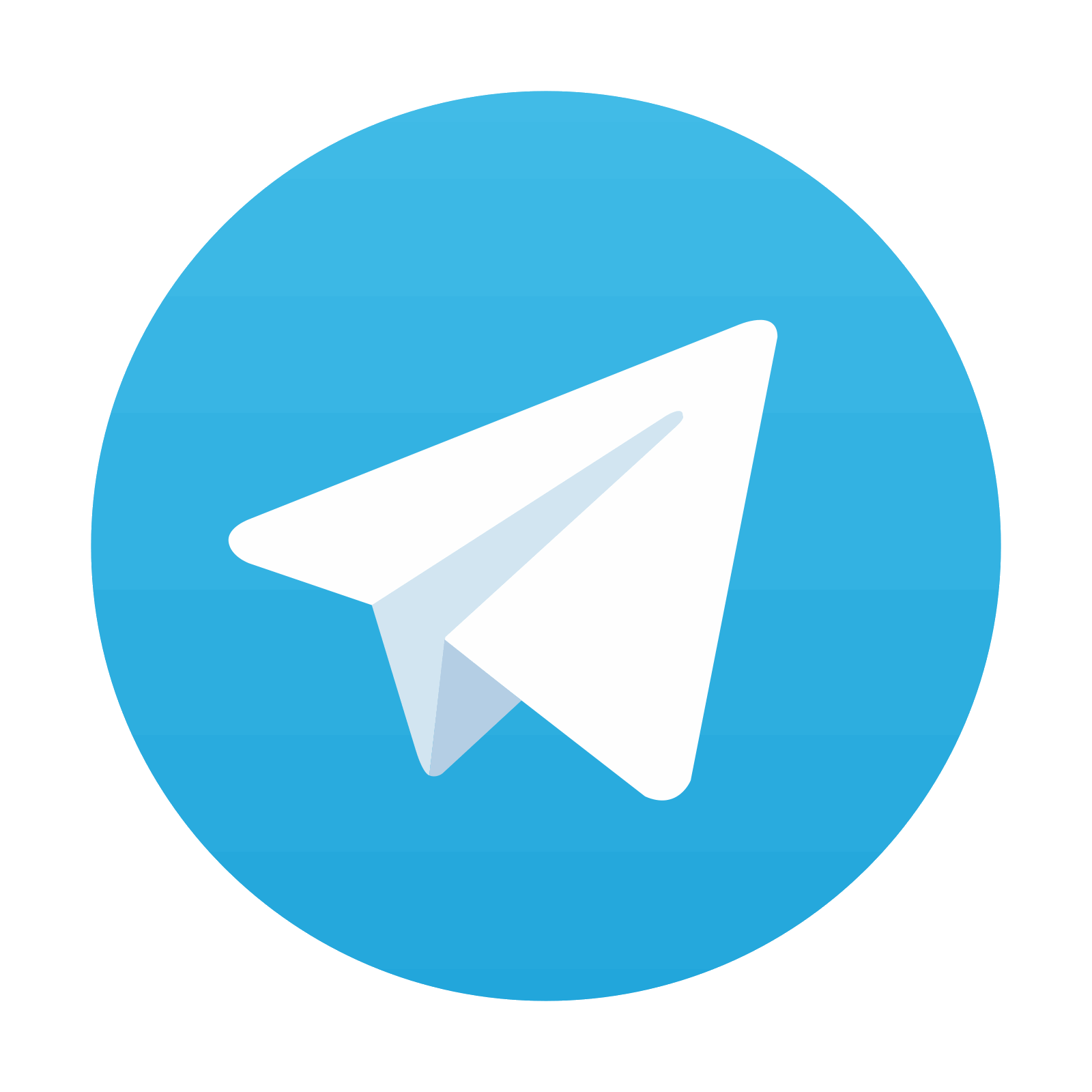
Stay updated, free articles. Join our Telegram channel
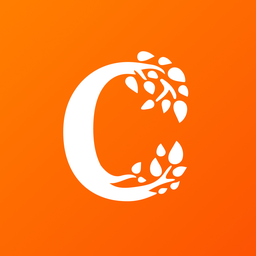
Full access? Get Clinical Tree
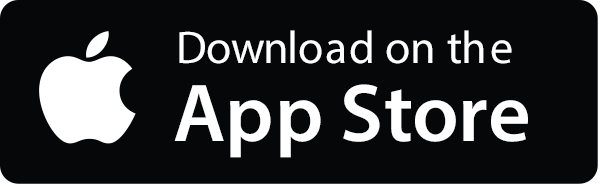
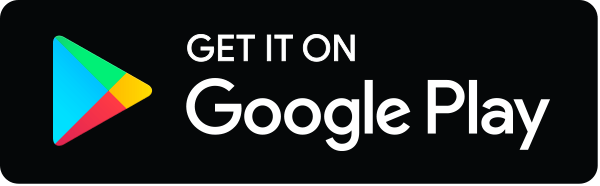